Advanced V-based materials for multivalent-ion storage applications
Abstract
Multivalent-ion batteries, as promising alternative or supplementary technologies to lithium-ion batteries, have increasingly attracted attention recently. Various advanced materials have been presented to pursue potential breakthroughs in energy and power. Among them, vanadium (V)-based materials benefiting from abundant resources, various polymorphs and valences, especially most with large interlayer spacings, are good candidates for multivalent-ion storage. However, limited by multiple inherent issues, e.g., strong electrostatic interactions, poor electronic conductivity, structure collapse or materials dissolution under battery operation, etc., various strategies have sprung many advanced materials and applications and also brought about new challenges that are in urgent need to clarify and summarize. Hence, advanced V-based compounds developed for multivalent-ion storage in the past few years are selectively summarized and systematically analyzed, including vanadium oxides and sulfides, vanadates, and V-based MXenes and phosphates. Not only crystal structures and electrochemical properties but also mainstream ion storage mechanisms are critically reviewed. Through analyzing the challenges accompanying multivalent-ion storage, potential opportunities are anticipated.
Keywords
INTRODUCTION
With ever-growing need of energy storage and electric vehicles, various rechargeable batteries have gained much attention. Among them, lithium-ion batteries (LIBs) are currently dominating the global market for mobile power sources, but issues from safety, cost, and limited resources have hindered their further development[1-3]. Multivalent-ion batteries, such as Mg2+, Ca2+, Zn2+, Al3+, etc., are attracting more and more interest due to their relatively high safety, considerable resource reserves, and good environmental friendliness[4-8]. However, multivalent ions carry more charges per ion than monovalent ions, which means a much stronger Coulomb interaction resulting in sluggish kinetics for ion diffusion[9]. Therefore, compared with monovalent-ion storage, it is much more crucial to search for suitable insertion hosts for efficient multivalent-ion storage.
There are many candidate electrodes, e.g., some layered oxides, Prussian blue analogs, organic compounds, etc., appropriate for multivalent-ion storage, but they are generally challenged by issues such as poor cycling stability, inherent low capacity, severe dissolution, and so forth[10]. Among them, vanadium (V)-based compounds with abundant valences and rich resource reserves guarantee high theoretical capacity and cost-effective scale-application prospects[11,12]. Meanwhile, changeable V-O coordination chemistry of VO4 tetrahedra, VO5 triangular bipyramidal/square pyramidal, and VO6 aberrant/ortho-octahedral and types of polymorphs and microstructures such as laminar, three-dimensional (3D) tunneling, chain, rock-salt structures, etc., also offer a wide structure regulation freedom[12]. Crucially, most V-based compounds exhibit large interlayer spacings conducive to fast insertion/extraction of various multivalent ions. However, issues such as active material loss due to dissolution also occurred in some V-based compounds similarly[13]. Complex reaction mechanisms and low average operating voltage are also occasional challenges. Overall,
OVERVIEW AND CATEGORIES
Before introducing the Mg2+, Ca2+, Al3+ and Zn2+ multivalent-ion storage performance, various categories of V-based materials and their structures are summarized below. For simplification, they are generally divided as vanadium oxides (VOx), vanadium sulfides (VSx), vanadates (MVOx), V-based phosphates (MVPO4), and V-based MXenes (VXenes), respectively.
Vanadium oxides
Vanadium oxides show different valences of V from 0.4 to 5, forming a variety of symmetries such as triclinic V4O7, V8O15, V7O13, and V6O11, monoclinic VO2 and V2O4, orthorhombic V4O9, tetragonal VO0.2, VO1.27 and V2O5, and cubic VO0.9 and VO. They can typically be treated as corner-, edge-, or face-sharing
V2O5
V2O5, with V at the highest oxidation state of +5 which means a relatively larger charge storage capability, usually crystallizes into four polymorphs, i.e., orthorhombic, monoclinic, tetragonal, and orthorhombic. Among them, α-V2O5 is the most common polymorph with V-O square pyramid coordination forming a lamellar structure by co-orientation or co-angulation [VO5] polyhedra[18]. The structure (Space group: Pmnm, a = 111.150 Å, b = 3.563 Å, and c = 4.370 Å) is usually thermodynamically stable[19]. It can be easy to regulate the weakly bonded lamellar structure to achieve fast insertion/extraction of different metal ions. However, the poor conductivities of both ions and electrons and intense host-guest interaction readily lead to significant volume change and easy polarization when V2O5 is used as a battery cathode. Thus, it is still difficult to achieve reversible and fast ion storage in pure V2O5[20].
VO2
VO2 can crystallize into more than a dozen phases, e.g., thermodynamically stable monoclinic VO2(M),
V2O3
V2O3 usually exhibits a rhombic corundum structure, characteristic of edge-sharing [VO6] octahedra packed into a 3D tunnel structure, favorable for ion intercalation[25]. The structure belongs to the
V6O13
V6O13 consists of alternating single- and double-twisted [VO6] octahedral layers in a jagged arrangement containing mixed-valence V5+/V4+[23,27]. V6O13 single- and double-twisted [VO6] octahedral layers shared corners, showing a 3D open frame structure[28]. Based on valence bonding and calculations, only some of the vanadium sites in the bilayer show V5+ properties, while V4+ occupies the remaining vanadium sites[23].
V3O7
V3O7 is of mixed-valence V5+/V4+ with an atomic ratio of 2:1, resulting in superior electrochemical properties compared to other vanadium oxides[12]. The crystal cell of V3O7 contains 36 vanadium atoms, 12 in octahedra and 24 in pentacoordination[18]. Its hydrate V3O7·H2O has a lamellar structure, consisting of V3O8 layers stacked along the a-axis with [VO6] octahedral and [VO5] square pyramid coordination by sharing corner/edge, and the H2O molecules are distributed on both sides of the V3O8 layer. Adjacent layers are usually interconnected by hydrogen bonds, providing a buffer space due to the vibration of hydrogen bonds[29]. Therefore, during ion insertion/extraction, large lattice distortion can easily occur without destroying the crystal structure[30]. Compared to orthorhombic V2O5, V3O7·H2O has a much larger layer spacing and is usually crystallized in a one-dimensional nanostructure[12].
Vanadium sulfides
Vanadium sulfide is available in many forms, e.g., VS2, VS4, V2S3, VS, V2S5, V3S, and V6S. Among them, VS2 and VS4 are usually used in batteries[31]. Their graphene-like structures afford large layer spacings, facilitating cation intercalation and diffusion. Vanadium atoms in VS2 and VS4 exhibit the same oxidation state, while sulfur atoms appear as S2- monomers in VS2 and S22- dimers in VS4, leading to entirely different physicochemical and electrochemical properties[32]. However, sulfides of vanadium are apt to be oxidized, especially when staying for a long duration in air atmosphere. This general phenomenon suggests that stringent conditions and atmosphere are essential for preparing or storing vanadium sulfide.
VS2
VS2 exhibits a lamellae structure similar to graphite. Each vanadium atom is linked to six sulfur atoms through covalent bonds, forming an S-V-S sandwich layer [Figure 3A] and weakly interlayer van der Waals bonding. The large interlayer spacing of 5.76 Å facilitates rapid ion diffusion and maintains structural integrity during repetitive cycling, making VS2 a promising candidate for pseudocapacitance[33]. Compared to vanadium oxides, weakened electrostatic interactions in VS2 result in a lower diffusion barrier for the cations, enabling reversible ion insertion/desertion. Additionally, VS2 has good electrical conductivity, which, together with a low ion diffusion barrier, contributes to good performance for metal-ion batteries.
Figure 3. Structures of (A) VS2, (B) VS4, (C) NaV3O8, (D) MgV2O4, (E) NH4V3O8, and (F) VOPO4. (G) Illustration of selectively etching process to synthesize V2CTx MXene. Reproduced with permission[63]. Copyright 2017, American Chemical Society.
VS4
VS4 consists of parallel and one-dimensional atomic chain-like structures with bonding V4+ and S22- dimer [Figure 3B], and the S22- dimer affords enough ion storage sites. The open channels with a chain spacing of 5.83 Å favor the diffusion and storage of cations. However, pure VS4 usually exhibits poor cycling performance and severe capacity degradation due to severe polarization, poor electron conductivity, and large volume expansion[34,35]. Various VS4 nanostructures, such as nanoribbons, nanorods, nanocones, and nanosheets, have been synthesized and assembled into microspheres or layered structures as electrode materials for batteries to solve the above problems[36,37].
Vanadates
Vanadates usually show better electrochemical performance than vanadium oxides due to structure optimization effects of extra metal ions. Similar to Zn0.25V2O5[38], many vanadates are made by inserting different cations into vanadium oxides, a strategy that preserves the rich chemical valence of the original vanadium oxide. Insertion of cations also increases the internal spacing of vanadium oxide, thus effectively mitigating the capacity loss. Furthermore, cations serve as pillars between layers, which prevents relative slip between adjacent V-O and supports both layers, stabilizing the V-O structure and avoiding vertical collapse[39]. According to the type of cations, vanadates can be divided into alkali metal and alkaline earth metal vanadate, transition metal vanadate, and other vanadates. Most vanadates were prepared using the hydrothermal method, so insertion of metal ions is usually accompanied by water molecules, forming vanadates with some crystallized water.
Alkali metal vanadates
LiV3O8 usually demonstrates good Li-storage performance[40]. Structures of NaV3O8 and LiV3O8 are similar, consisting of edge- or corner-sharing [VO6] octahedra layers, and Li+ and Na+ are located between the layers [Figure 3C]. Due to the large radius of K+, it is impossible to form a similar structure. Differently, V2O8 units and VO6 octahedra share edges and corners in KV3O8[41], two VO5 square pyramids are connected by a VO6 octahedron to form a (V3O12)n chain stacked along the b-axis. Then, another reversed (V3O12)n chain connects the above (V3O12)n chains to form a KV3O8 layer along the b-axis[42]. Li3V6O13 is also a typical alkali metal vanadate. It consists of octahedral [VO6] and triangular pyramidal [VO5] units shared on both sides, resembling strings and bands arranged along (110) crystal planes. Layer-structured octahedra and tetrahedra are connected by interstitial Li+[43], forming a square cone ligand with five O atoms without occupying insertion sites of V6O13.
Alkali earth metal vanadates
MgV2O4, MgxV2O5, CaV6O16·2.8H2O, etc., are common alkaline earth metal vanadates reported for multivalent-ion storage. MgV2O4 consists of [VO6] octahedra and [VO4] tetrahedra, and Mg2+ can be inserted at the tetrahedral sites of the spinel oxide [Figure 3D]. The AV2O4-typed spinel (A = magnesium (Mg), calcium (Ca), etc.) is an attractive structure with which electrodes often exhibit abundant 3D channels, good crystal stability, tunable atomic scale structure, and suitable operating voltage[44]. The structure of MgxV2O5·nH2O is similar to bilayer V2O5 that consists of [VO6] octahedra as the basic layer with Mg2+ or hydrated Mg2+ inserted between the layers[45]. The biotite talc CaV6O16·2.8H2O consists of a reconstructed α-V2O5 structure with layers comprising [VO5] square pyramids and [VO6] octahedra. The interlayered Ca2+ and water molecules raised the layer spacing from 4.37 to 8.10 Å and stabilized the interlayer structure[46].
Transition metal vanadates
Most ions of transition metals, e.g., Ag+, Fe2+/Fe 3+, Zn2+, Co2+, Cu2+, Mn2+/Mn 3+, Ni2+, etc., readily combine vanadium oxides to form the corresponding vanadates. AxV2O5·nH2O (A = Zn, manganese (Mn), Ni, Co, etc.) exhibits a layered structure similar to V2O5, with alternate [VO6] octahedral layer and hydrated cations between the layers[38,47]. ZnV2O4, akin to spinel MgV2O4, consists of [VO6] octahedra and [VO4] tetrahedra. Since the electrochemical performance of low-valent V-based oxides in aqueous solution is limited, researchers tend to oxidize ZnV2O4 electrochemically in the first few cycles, which leads to superior electrochemical performance[48]. Other transition metal vanadates include FeVO4[49], Cu3V2O7(OH)2·H2O[50], CuV2O6[51], ZnV6O16·8H2O[52], Fe2V4O13[53], etc., which are also reported efficient for multivalent-ion storage.
Other vanadates
Besides, aluminum (Al)-based and NH4-based vanadates are relatively less studied. Actually, with a molecular weight relatively lower than that of transition metal vanadates, they generally deliver much larger specific capacities. Besides, insertion of Al3+ or NH4+ also makes the interlayer spacings larger and the ion conductivity higher, favoring the diffusion of metal ions[54]. With edge-shared twisted [VO6] octahedra, the monoclinic NH4V4O10 exhibits a stable bilayer structure. The NH4+ ion tends to act as a backbone cation to stabilize the structure, preventing severe volume changes during insertion of guest ions[55]. Hollow spheres of H11Al2V6O23.2 with a bilayer structure and low crystallinity showed little lattice distortion and good long-term cycling stability[56]. Crystallized NH4V3O8 has a twisted zigzag layer structure consisting of VO5 square cone units and twisted [VO6] octahedra parallel to the (00l) plane, with NH4+ ions between the layers connecting with oxygen atoms through hydrogen bonding [Figure 3E][57].
V-based phosphates
A prominent feature of V-based phosphates is the output voltage higher than that of vanadates or vanadium oxides due to the inductive effect of the (PO)43- group[58]. Moreover, V-based phosphates also exhibit good structure stability and fast ion diffusion[58,59]. VOPO4, Na3V2(PO4)2F3, Li3V2(PO4)3, Na3V2(PO4)3, etc., are common explored V-based phosphates. VOPO4 displays a typical layered crystal structure with vertex-sharing VO6 octahedra and PO4 tetrahedra in a 1:1 ratio [Figure 3F][60]. Hydrothermal synthesized VOPO4 tends to form the hydrate VOPO4·nH2O, which easily decomposes into VOx during cycling, so the cycling performance is poor[61]. The 3D framework of Na3V2(PO4)3 cathode consists of strongly bonded tetrahedral
V-based MXenes
MXenes (M and X stand for an early transition metal and C/N elements, respectively) represent a series of two-dimensional (2D) inorganic compounds comprising several atomic layers of transition metal carbides, nitrides, or carbon-nitrides. They are typically produced by selectively removing metal ions in the A layer in the MAX phase with a combination of HF or LiF/HCl aqueous solution as etchants [Figure 3G][63]. Meanwhile, they also exhibit extraordinary physical, chemical, and electrochemical properties, such as hydrophilic surfaces, ultra-high electrical conductivity, and accordion-like laminate structure, making MXenes a good candidate to form a series of functional composites[64]. The primary four types of MXenes are titanium, niobium, vanadium, or molybdenum-based. Among them, most studies are Ti-based MXenes with large interlayer spacings, and abundant active sites allow for fast insertion/extraction of various guest ions[65]. However, the non-environmentally friendly preparation mostly involves harmful and hazardous HF, limiting the application of MXenes. Currently, V2CTx (Tx representing different surface functional groups) is the widely used V-based MXenes in multivalent-ion batteries[63], which undergoes multi-electron redox reactions. Due to its low valence of V (+2 and +3), it usually exhibits a relatively low capacity. To enhance the ion storage capability, in situ electrochemical activation was used to increase the valence state of V in
MULTIVALENT-ION STORAGE APPLICATION
Non-aqueous batteries
Batteries with non-aqueous electrolytes usually exhibit a much wider electrochemical window and higher energy density than aqueous ones. However, active metal anodes in organic electrolytes tend to form insulative and passivated interphases, resulting in sluggish ion transport. It is a significant obstacle to reversible plating/stripping processes for Mg-/Ca-metal batteries. Differently, the ion diffusion rate in aqueous electrolytes is faster than in organic ones due to the fact that water has a charge shielding effect which can reduce the polarization of ions to the host lattice[69]. Therefore, small amounts of water are sometimes introduced into the organic electrolytes to reduce polarization and improve the reaction kinetics by obtaining a charge-shielding effect or converting metal ions into low-polarized solvated ions.
Mg-metal batteries
With many virtues of Mg, such as rich natural abundance, high capacity, low redox potentials, etc., Mg-metal batteries have been intensively explored. Aurbach et al. reported the first highly reversible rechargeable magnesium battery system using Mo6S8 as the cathode in 2000[69]. However, the low capacity and discharge voltage of Chevel-phase Mo6S8 limit further development. So far, many electrode materials have been explored, such as transition metal sulfides[69], transition metal oxides[70], polyanionic compounds[71], and organic materials[72] for Mg-storage. Among them, V-based materials, with abundant valence states and unique layered structures or open backbones favoring reversible insertion/extraction of considerable Mg2+ ions, have been increasingly attractive. However, strong Mg-host interactions due to inherent divalent charge and large radius cause severe ion polarization, which is one of the main reasons hindering the application of V-based compounds.
To alleviate the relevant issues of inherent small layer spacings and strong electrostatic interactions, strategies, such as pre-intercalating large organic ions, adding electrolyte additives or surfactants, doping, etc., have been frequently investigated. Through intercalating large organic cation of C10H22N+ in the first discharge, the interlayer spacing of VS2 was significantly enlarged, raising the diffusion coefficient of Mg2+ to 10-10-10-12 cm-2 s-1[73]. Thus, the corresponding cathode of VS2 nanosheets achieved a large capacity of
In most cases, Mg-storage in vanadium sulfides is based on the Mg2+ intercalation mechanism, but intercalation of complexion ions, e.g., MgCl+, has also been frequently disclosed. For example, through both theoretical and experimental studies, Pei et al. found that MgCl+ reversibly intercalated into/deintercalated out of VS4@reduced graphene oxide (rGO) rather than Mg2+ when 0.25 M [Mg2Cl3]+[AlPh2Cl2]-/tetrahydrofuran was used as the electrolyte, contributing to 268.3 mAh g-1 at 50 mA g-1[77]. Zhu et al. also observed reversible intercalation/deintercalation of MgCl+ in VS4 nanosheets /carbon-coated Ti3C2-MXenes hybrid cathodes[68]. The presence of V-C bonding proved a strong coupling between VS4 and Ti3C2, and this unique layered nano-microstructure improved the accessibility of electrolytes, which reduces resistance of charge transfer. So, the hybrid delivered 492 mAh g-1 at 50 mA g-1. After 900 cycles, it also held 80% capacity retention at 0.5 A g-1. In addition, co-intercalation of Mg2+ and MgCl+ also happened to some vanadium sulfides. For example, 2-methylhexanamine in situ intercalated VS2 with a large layer spacing of 9.93 Å reversibly intercalated/deintercalated Mg2+ and MgCl+, as shown in the corresponding XPS and EDS characterization [Figure 4A-C][78]. A polyvinylpyrrolidone/VS4 composite was also found to simultaneously intercalate Mg2+ and MgCl+[79].
Figure 4. XPS spectra of (A) Mg 2s, (B) Cl 2p, and (C) EDS spectra for expanded VS2 electrodes. Reproduced with permission[78]. Copyright 2019, Wiley-VCH GmbH. (D) Charge-discharge curves (A = Li, Na, K) and (E) cycling performance of A-V3O8 (A = Li, Na, K) at 100 mA g-1, and (F) spacing change of NaV3O8 at different charge-discharge stages. Reproduced with permission[84]. Copyright 2019, Elsevier. (G) Formation of CaV6O16·2.8H2O and (H) Ca-storage in CaV6O16·2.8H2O. Reproduced with permission[178]. Copyright 2022, Wiley-VCH GmbH. (I) Cycling performance of Zr-NH4V4O10 at 0.2 A g-1. Reproduced with permission[92]. Copyright 2022, Elsevier.
Compared to vanadium sulfides, the high electronegativity of oxygen leads to higher ionic character of V-O bonding in the oxides, and the strengthened bonding usually raises the electrochemical potential for metal-ion intercalation. Moreover, higher voltage and lower molecular weight will increase the specific energy. For example, monodispersed V2O5 hierarchical spheres delivered good performance of 190 mAh g-1 at
However, pure V2O5 is severely confined for Mg-storage because of poor ion and electron transport processes. It is efficient to improve the conductivity by intercalating electron conductive organics. For example, a 2D organic-inorganic superlattice with alternately arranged monolayered V2O5 nanosheets and polyaniline (PANI) monolayers exhibited a Mg-storage capacity of 270 mAh g-1 at 100 mA g-1, far superior to only 102 mAh g-1 of pure V2O5 under the same testing conditions. Benefiting from the π-π conjugated chains, monolayer PANI not only served as pillars to enlarge layer spacings but also functioned as electron conducting pathways and active sites for Mg-storage[81]. Another V2O5/PEDOT (3,4-ethylene dioxythiophene) hybrid cathode achieved 348.3 mAh g-1 at 100 mA g-1 due to a widened interlayer spacing of 19.2 Å about 4.37 times that of pure V2O5[82]. Besides, it is also effective to improve the kinetics of V2O5 by introducing oxygen vacancies. An O-vacancy riched Ti-V2O5-x with a honeycomb structure exhibited an impressive electronic conductivity six times higher than that of pure V2O5. Due to the improved kinetics, the O-vacancy riched electrode delivered a Mg-storage capacity of 245.4 mAh g-1 at 100 mA g-1, except for 79.6% retention after 400 cycles[83].
Pre-intercalation of metal ions into vanadium oxides also enhances the Mg-storage performance. For example, V3O8 with hydrothermally intercalated Li+, Na+ and K+ ions delivered different Mg-storage capacities of 252.2, 204.16 and 37.56 mAh g-1 at 100 mA g-1, respectively, [Figure 4D] except for the capacity retention of 42.2%, 85.78%, and 88.6% [Figure 4E], respectively, after 30 cycles[84]. The V-O layer spacing at different charging and discharging stages does not recover to be the same as the initial [Figure 4F]. Some Mg2+ may remain in the interlayers and gradually accumulate during the cycling process, resulting in poor cycling performance. Other metal-ion intercalated vanadium oxides, e.g.,
Although interlayer structures of vanadium oxides can be stabilized with intercalated metal ions, the effects of space charge repulsion and occupied active sites by pre-intercalated ions will reduce initial capacities. Differently, water molecules can effectively widen the interlayer spacings and buffer the charge repulsion effect between guest ions and elements from the host structures. There are two common strategies to introduce water molecules. One is in-situ formation of active materials with crystal water in an aqueous solution, and the crystal water acts as both interlayer support and charge shielding layers. The hydrothermally synthesized NaV3O8·1.69H2O nanoribbons exhibited a Mg-storage capacity of 110 mAh g-1 at 10 mA g-1, which decays rapidly due to fast Mg consumption from leaching crystal water[85]. Second, trace amounts of water in organic electrolytes can be more ion-conductive. Meanwhile, it does not lower the electrochemical stabilization windows. For example, NaV8O20·nH2O in mixed solvents of tetramethylene glycol dimethyl ether (TEGDME)/water (4:1 by volume) delivered much better performance than that in pure TEGDME (351 mAh g-1vs. 169 mAh g-1 at 0.3 A g-1), except for a wide window voltage of 3.9 V[86].
As to V-based phosphates, pre-intercalation of small molecules of H2O or organics was also explored to improve the Mg-storage performance. For example, water or aniline molecules enhanced the diffusion kinetics of VOPO4 cathodes due to widened interlayer spacings. Benefiting from a fast MgCl+ intercalation mechanism, the cathode delivered 310 mAh g-1 at 50 mA g-1 and 192 mAh g-1 at 0.1 A g-1 even after
Performance comparison of V-based materials for multivalent-ion batteries
Materials | Application | Electrolyte | Capacity (mAh g-1) | Cycle performance |
NaV6O15[179] | MIB | 0.5 M Mg(ClO4)2/AN | 137 (0.05 A g-1) | 80%, 10 mA g-1 (100 cycles) |
Mg0.3V2O5·1.1H2O[16] | MIB | 0.3 M Mg (ClO4)2/AN | 164 (0.1A g-1) | 80%,2 A g-1 (10,000 cycles) |
FeVO4[49] | MIB | 0.3 M Mg(ClO4)2/AN | 270 (0.5 A g-1) | 85%,1 A g-1 (10,000 cycles) |
H11Al2V6O23.2[180] | MIB | 0.3 M Mg(ClO4)2/AN | 165 (0.1 A g-1) | 87%,1 A g-1 (3,000 cycles) |
Li3V2(PO4)3[88] | MIB | 0.3 M Mg(ClO4)2/PC | 124 (0.1 A g-1) | 80%,0.5 A g-1 (300 cycles) |
VS2[73] | MIB | 0.4 M APC-PP14Cl/THF | 214 (2 A g-1) | 78%,1 A g-1 (300 cycles) |
Mn0.04V2O5·1.17H2O[181] | MIB | 0.3 M Mg(ClO4)2/AN | 145 (0.05 A g-1) | 82%,1 A g-1 (10,000 cycles) |
CaV6O16·2.8H2O[46] | CIB | Ca(TFSI)2/G2 | 134.7 (0.1 A g-1) | 75%,0.1 A g-1 (50 cycles) |
Mg0.25V2O5·H2O[182] | CIB | 0.8 M Ca(TFSI)2 in carbonate | 120 (0.02 A g-1) | 86.9%,0.5 A g-1 (500 cycles) |
Ca0.28V2O5·H2O[90] | CIB | 0.5 M Ca(ClO4)2/PC | 142 (0.01 A g-1) | 74%,0.03 A g-1 (50 cycles) |
K2V6O16·2.7H2O[114] | CIB | 5 M Ca(NO3)2 | 114 (0.02 A g-1) | 78.5%,0.05 A g-1 (100 cycles) |
NH4V4O10[92] | CIB | 0.25 M Ca(TFSI)2/PC | 77 (0.05 A g-1) | 89%,0.2 A g-1 (500 cycles) |
Ca0.26V2O5·H2O[89] | CIB | 0.8 M Ca(TFSI)2 in carbonate | 196 (0.02 A g-1) | 93.6%,1 A g-1 (2,500 cycles) |
FeV3O9·1.2H2O[183] | CIB | 0.5 M Ca(ClO4)2/AN | 96 (0.2 A g-1) | 79%,0.2 A g-1 (400 cycles) |
Li2V6O13[96] | AIB | [EMIm]Cl:AlCl3 = 1:1.3 | 159 (0.1 A g-1) | 73%,0.05 A g-1 (300 cycles) |
VS4[98] | AIB | [EMIm]Cl:AlCl3 = 1:1.3 | 408 (0.1 A g-1) | 39%,0.5 A g-1 (500 cycles) |
V2O5[184] | ZIB | 2 M ZnSO4 | 425 (0.3 A g-1) | 78.5%,3 A g-1 (200 cycles) |
VO2[185] | ZIB | 3 M Zn(CF3SO3)2 | 280 (0.1 A g-1) | 86%,3 A g-1 (5,000 cycles) |
V3O7[186] | ZIB | 2.5 M Zn(CF3SO3)2 | 233 (0.2 A g-1) | 96.2%,2 A g-1 (1,120 cycles) |
V6O13[28] | ZIB | 3 M Zn(CF3SO3)2 | 360 (0.2 A g-1) | 92%,4 A g-1 (2,000 cycles) |
V2O3[140] | ZIB | 3 M Zn(CF3SO3)2 | 196 (0.1 A g-1) | 81%,5 A g-1 (30,000 cycles) |
VS2[127] | ZIB | 1 M ZnSO4 | 187 (0.1 A g-1) | 80%,2 A g-1 (2,000 cycles) |
VS4[130] | ZIB | 2 M Zn(CF3SO3)2 | 265 (0.25 A g-1) | 93%,5 A g-1 (1,200 cycles) |
NH4V4O10[187] | ZIB | 3 M Zn(CF3SO3)2 | 298 (0.1 A g-1) | 89%,2 A g-1 (2,000 cycles) |
LiV3O8[188] | ZIB | 3 M Zn(CF3SO3)2 | 298 (1 A g-1) | 85%,5 A g-1 (4,000 cycles) |
Na0.33V2O5[189] | ZIB | 3 M Zn(CF3SO3)2 | 367 (0.1 A g-1) | 93%,1 A g-1 (1,000 cycles) |
Mg0.2V2O5[67] | ZIB | 3 M Zn(CF3SO3)2 | 346 (0.1 A g-1) | 83.7%,5 A g-1 (10,000 cycles) |
ZnV3O8[190] | ZIB | 3 M Zn(CF3SO3)2 | 294 (0.1 A g-1) | 74.6%,2 A g-1 (1,200 cycles) |
Fe2V4O13[53] | ZIB | 2 M Zn(CF3SO3)2 | 380 (0.2 A g-1) | 83%,10 A g-1 (1,000 cycles) |
Na3V2(PO4)2F3[191] | ZIB | 2 M Zn(CF3SO3)2 | 60 (0.2 A g-1) | 95%,1 A g-1 (4,000 cycles) |
Ca-metal batteries
The lower reduction potential of Ca (-2.87 V vs. SHE) than Mg allows its metal batteries to deliver much higher voltages. Meanwhile, the lower charge density and polarization also contribute to better diffusion kinetics. However, various vanadium oxides suffered severe structural degradation and collapse during ion insertion/extraction. The derivatives with pre-intercalated metal ions, e.g., AxV2O5·nH2O, where A stands for metal ions, exhibited good structural stability in Ca-storage. At a testing temperature of 50 °C, reversible capacities of 142.4, 109.8, and 86.6 mAh g-1 were obtained in Mg0.25V2O5·H2O, Ca0.26V2O5·H2O, and
In addition to oxide derivatives with pre-inserted metal ions, vanadates, such as CaV6O16·2.8H2O[46], are also good candidates for Ca-storage. CaV6O16·2.8H2O showed capacities of 175.2 mAh g-1 at 50 °C and
Al-/Zn-metal batteries
Rechargeable aluminum batteries (RABs) have been intensively focused due to their high safety and rich aluminum abundance[93]. However, they still face many issues, such as severe corrosion of liquid electrolytes, significant volume change, low discharge voltage, poor reversibility, and so on[94,95]. Wang et al. reported that a FeVO4@ PANI nanoribbon composite held 300 mAh g-1 after 300 cycles at 0.3 A g-1[93]. Besides, it achieved 268.6 mAh g-1 after 200 cycles, even at a low temperature of -10 °C. A pre-lithium vanadium oxide derivative of Li2V6O13 attained 161.6 mAh g-1 at 50 mA g-1 after 300 cycles, far superior to that of pristine
Metallic zinc is also a safe anode. However, the research on Zn-metal batteries in organic electrolytes is limited due to lower voltages and capacities. A flower-like NH4V4O10 attained a capacity of 486 mAh g-1 at 0.1 A g-1 in 1 M Zn (ClO4)2/propylidene carbonate (PC) electrolyte [Figure 5A], which displayed little capacity decay at 10 A g-1 for 3,000 cycles [Figure 5B][99]. With the same electrolyte, a paper-like electrode with perfectly aligned Na2V6O16·3H2O nanoribbons exhibited 216 mAh g-1 at 0.5 A g-1, and 167 mAh g-1 was still attained at 5 A g-1 after 5,000 cycles[100]. Besides, a composite with V2O5·1.6H2O/Ti3C2 MXenes heterostructured nanosheets delivered 205.5 mAh g-1 at 0.1 A g-1 in triethyl phosphate electrolyte with a trace of water, and capacity retention of 78.6% was obtained at 0.5 A g-1 after 4,000 cycles[101]. The performance is also compared in Table 1.
Figure 5. (A) Rate and (B) long-term cycling performance of Na2V6O16·3H2O. Reproduced with permission under the terms of the Creative Commons Attribution[99]. Copyright 2020, the Author(s), Springer Nature. (C) Working mechanism and (D) cycling performance of VS2-GO. Reproduced with permission[103]. Copyright 2018, Elsevier.
Hybrid-ion batteries
Considering the limitations of single-ion storage, e.g., safety issues and high cost of lithium, high polarization of Mg2+, hybrid-ion storage has attracted increasing attention in recent years[102]. For example, in a Mg/Li hybrid electrolyte, Li+ dominates the cathode insertion because the diffusion rate of Li+ in the cathode is much larger than that of Mg2+. At the discharge, Mg2+ ions are dissolved from the Mg anode, while Li+ ions are inserted into the cathode [Figure 5C][103]. Meanwhile, in the charge process, the situation is just the opposite. A hybrid Mg-Li-ion battery combines the advantages of a Mg anode without dendrite deposition and a fast lithium insertion cathode, making it a better alternative to LIBs for power storage[104].
Layered V-based compounds are good ion intercalation hosts due to their large specific capacity and multi-electronic reactions. For example, the hybrid batteries of Mg2+/Na+ and Mg2+/K+ with VS2 cathodes were also explored. It was observed that ions of Li+, Na+, or K+ could be co-inserted with Mg2+ into VS2. Differently, co-insertion of Mg2+/Li+ or Mg2+/K+ led to the collapse of VS2, while Mg2+/Na+ reversibly co-intercalated into VS2, contributing to a capacity of 170 mAh g-1 at 0.1 A g-1 and 96.5% retention after 1,000 cycles[105]. A
Aqueous batteries
Compared with organic electrolytes, aqueous electrolytes, benefiting from good conductivity, low cost, high safety, etc., have attracted intensive attention in energy storage[108]. The multivalent metal-ion storage of V-based compounds in aqueous electrolytes is discussed in the following.
Mg-/Ca-ion batteries
The available electrode materials for aqueous Mg-ion batteries have faced issues such as limited storage capability due to sluggish Mg-ion diffusion kinetics, easy structure degradation accompanying Mg-ion intercalation resulting from large volume effect and dissolution of active materials, etc. Therefore, relevant references are much less than those about non-aqueous batteries. Zhang et al. used VO2 as an anode and
Figure 6. (A) δ-MnO2//MgCl2 (aq.)//VO2 operation from 25 to -50 °C and (B) molecule dynamic simulation of water and MgCl2 electrolyte. Reproduced with permission[111]. Copyright 2023, Elsevier. (C) Synthesis of FeVO4/C and (D) relevant cycling performance. Reproduced with permission[112]. Copyright 2017, Wiley-VCH GmbH.
Since rechargeable calcium batteries based on organic electrolytes are severely limited in cycling performance and kinetics, Ca-ion batteries with aqueous electrolytes would be an exciting alternative to avoid issues faced by Ca deposition in organic electrolytes and to extend the choice of active materials. An anode material of CaV6O16·7H2O synthesized by a molten salt method exhibited an initial discharge capacity of 208 mAh g-1 at 12.5 mA g-1, and a high retention of 97% was obtained after 200 cycles. CVs Cyclic voltammetry curves under different pH values of 2.3 and 10 confirmed that Ca2+ intercalation rather than H+ dominated the energy storage mechanism[113]. A hydrothermally synthesized K2V6O16·2.7H2O cathode initially discharged 113.9 mAh g-1 at 20 mA g-1 in a three-electrode aqueous Ca-ion system and held 78.3% capacity after 100 cycles at 50 mA g-1[114]. The comparison of these performances is also summarized in Table 1.
Al-ion batteries
With large theoretical capacity, abundant aluminum resources, and high safety, aqueous aluminum ion batteries have been attractive recently. A layered LiV3O8 cathode material delivered 205 mAh g-1 in
Mn-ion batteries
Unlike Mg and Al metals with high redox potentials, metal Mn with lower redox potentials is a promising candidate material[119]. Furthermore, Mn has high abundance, good salt solubility, and a small ion radius[120]. All of these indicate that rechargeable aqueous Mn-ion batteries are feasible. However, there are almost no reports on Mn2+ carriers in battery research. A Mn0.18V2O5·nH2O cathode delivered 83.3 mAh g-1 at 5.0 A g-1 in 1 M Mn(CF3SO3)2 aqueous solution and held 86.7% capacity after 200 cycles at 5.0 A g-1[121]. Yang et al. used V2O5 as a cathode, sucrose as a water-splitting inhibitor, and sodium perchlorate (NaClO4) and glycine as electrolytes; a strong organic-inorganic interface is formed on Mn metal[119]. The assembled Mn||V2O5 battery delivers 180 mAh g-1 at 0.5 A g-1 and maintains approximately 100% capacity after 200 cycles at
Zn-ion batteries
Zinc has advantages such as high redox potential, high density, large theoretical volumetric energy density, low cost, and high content[122]. V-based compounds are ideal cathodes for aqueous Zn-ion batteries. The relevant salts used mainly include ZnCl2, ZnSO4, Zn(CF3SO3)2, etc. Among them, the use of ZnSO4 in
(1) Electrochemical performance
The large interlayer spacings of vanadium sulfides facilitate fast Zn2+ diffusion and intercalation. For example, VS2 delivered 159.3 mAh g-1 at 0.1 A g-1 in ZnSO4 electrolyte and held 81% capacity at 0.5 A g-1 after 200 cycles[126]. A much better performance of 187 mAh g-1 at 0.1 A g-1 and 85% retention after 2,000 cycles at 2 A g-1 was achieved when VS2 was used as a cathode[127]. A VS2/VOx [Figure 7C] heterostructure was reported to deliver 310 mAh g-1 with 75% retention after 3,000 cycles. Moreover, the working potential increased by 0.25 V compared with that of pure VS2 at 1 A g-1 [Figure 7D][128]. A VS2@N-C hybrid with enhanced reactivity and interfacial charge transfer by N-doping delivered 203 mAh g-1 at 50 mA g-1, and a retention of 97% was retained after 600 cycles at 1 A g-1[129]. In contrast, VS4 suffered severe volume changes and dissolution of polysulfides after Zn insertion. Specifically, it was initially converted to Zn3+x(OH)2V2O7 in the initial cycles, and Zn2+ was subsequently inserted into/ extracted out of the open framework structure reversibly. For example, a flower-like VS4/carbon nanotubes (CNTs) nanocomposite showed a capacity of 182 mAh g-1 at 0.25 A g-1 and 93% retention after 1,200 cycles at 5 A g-1[130]. Another VS4@rGO electrode delivered 180 mAh g-1 at 1 A g-1 with 93.3% retention after 165 cycles[131].
Vanadium oxides have a wide range of applications in aqueous Zn-ion batteries. V2O5 exhibits a theoretical capacity of 589 mAh g-1 based on V5+/V3+ redox, but the severe deformation accompanying Zn insertion/extraction readily leads to unstable cycling performance[132]. Proper content of water molecules in interlayers of VOx polyhedrons avails to shield strong Zn2+ host interaction and stabilize the host structure. For example, water molecules in V2O5·nH2O functioned as a buffer layer, weakening the effective charge of intercalated Zn2+, leading to good rate performance of 248 mAh g-1 even at 30 A g-1 [Figure 8A][133]. The cycling performance can also be improved with conductive support. A nano paper electrode comprised of V2O5 nanofibers and multiwalled CNTs held 168.5 mAh g-1 at 10 A g-1 for 500 cycles[134]. A nanocomposite with heterostructures of V2O5 nanosheets and Ti3C2Tx MXenes layer showed enhanced conductivity and robust structure and exhibited stable cycling performance for 5,000 cycles with 99.5% capacity retention at 10 A g-1[41].
Figure 8. (A) The discharge curves of V2O5·nH2O at 0.3-30 A g-1. Reproduced with permission[133]. Copyright 2017, Wiley-VCH GmbH. (B) Diffusion paths in V2O5 and Al-V2O5. Reproduced with permission[137]. Copyright 2022, Elsevier. (C) Cycling performances and (D) reaction mechanism of V2O3. Reproduced with permission[139]. Copyright 2021, American Chemical Society. (E) Energy storage mechanism in the Zn|| V2O3 cells. Reproduced with permission under the terms of the Creative Commons Attribution[140]. Copyright 2021, the Author(s), Springer Nature.
Oxygen vacancies usually enhance the conductivity and improve the performance. Dendrites of
Corundum-type V2O3 with unique channels and suitable pore size distribution shows fast insertion/extraction of Zn2+[138]. Oxygen-deficient carbon-coated V2O3 delivered 662 mAh g-1 at 0.2 A g-1 [Figure 8C] after turning into Zn0.4V2O5-m·nH2O during the first charge [Figure 8D][139]. Similarly, V-deficient V2O3 also delivered enhanced Zn-storage capability because vanadium-defect clusters could afford favorable intercalation sites for Zn ions, as revealed by calculations. In addition, intercalated Zn2+ at the V vacancies serves as doped heteroatoms, making the host structure more stable [Figure 8E][140].
V3O7 with mixed valences (V4+/V5+) provides more active sites[140]. A uniform and ultrafine V3O7·H2O nano-network delivered 481.3 mAh g-1 at 0.1 A g-1 [Figure 9A] and 85.4% capacity retention at 5A g-1 for
Figure 9. (A) Voltage profiles and (B) long-term cycling stability of V3O7·H2O cathode. Reproduced with permission[141]. Copyright 2019, Royal Society of Chemistry. Diffusion paths of Zn ions (C) with and (D) without water and (E) calculated diffusion barriers for paths in (C and D). Reproduced with permission[28]. Copyright 2019, Wiley-VCH GmbH. (F) Cycling performance of Zn//CO2-V6O13 and Zn//P-V6O13 at 2 A g-1 and (G) CO2 molecules modified layer structured material. Reproduced with permission[145]. Copyright 2021, American Chemical Society.
Similarly, V6O13 delivered 360 mAh g-1 at 0.2 A g-1, benefiting from reduced diffusion barriers of hydrated Zn2+ [Figure 9C-E][28]. A 3D nested structure of V6O13 cathodes even exhibited a capacity of 520 mAh g-1 at 0.5 A g-1 and 85.3% retention at 2 A g-1 after 1,000 cycles due to short diffusion depth and large surface[144]. The electrode of V6O13 with trapped CO2 molecules showed a capacity of 471 mAh g-1 at
Unlike other vanadium oxides, tunnel-structured VO2 showed enhanced structural stability, benefiting from shared corner and edge resistance to lattice shearing accompanying ion insertion/extraction[146]. However, low conductivity and instability in acidic aqueous solutions limited its ion storage capability and cycle performance. For example, a capacity of 610 mAh g-1 was achieved at 0.1 A g-1 by in situ electrochemical oxidation of VO2 nanorods to V2O5·nH2O[147]. Similar transitions were also observed for monoclinic VO2[148]. Besides V2O5·nH2O, VO2 could also be in situ converted into ZnV2O7, which showed 408.4 mAh g-1 at
Figure 10. (A) XRD characterization of the VOP cathode at different charge-discharge stages and (B) phase transition disclosed from the differential capacity curve at various concentrations. Reproduced with permission[162]. Copyright 2019, Wiley-VCH GmbH. (C) Cycling performance of Co0.247V2O5·0.944H2O at 4 A g-1. Reproduced with permission[151]. Copyright 2019, Wiley-VCH GmbH. (D) Charge-discharge curves of H11Al2V6O23.2 at 0.1-5.0 A g-1. Reproduced with permission[56]. Copyright 2020, Elsevier. (E) Cycling performance of (NH4)0.38V2O5/CNTs paper electrode. Reproduced with permission[160]. Copyright 2021, Elsevier. (F) Typical charge-discharge curves of NH4V3O8·0.5H2O and PANI- NH4V3O8·0.5H2O electrodes at 1 A g-1. Reproduced with permission[57]. Copyright 2022, Elsevier. Typical charge-discharge curves of NH4V4O10 at (G) 0.2-1.4 V, (H) 0.2-1.6 V, and (I) 0.2-1.8 V. Reproduced with permission under the terms of the Creative Commons Attribution[161]. Copyright 2023, the Author(s), Wiley-VCH GmbH.
Vanadates are also good candidates for Zn-storage. The layered LiV3O8 discharged 200 mAh g-1 at
The presence of hydrogen bonding between NH4+ and V-O layers makes a stable structure in ammonia vanadate, resulting in excellent long-term cycling stability[159]. For example, ultrathin (NH4)2V10O25·8H2O nanoribbons, with large interlayer spacings of 1.045 nm favoring fast Zn2+ diffusion, achieved 228.8 mAh g-1 at 100 mA g-1 and 90.1% retention at 5 A g-1 after 5,000 cycles[54]. A binder-free cathode of (NH4)0.38V2O5 nanoribbons delivered 465 mAh g-1 at 100 mA g-1, and the retention was 89.3% after 500 cycles
V-based phosphates showed high discharge plateaus due to strong inductive effect of PO43- and represented a type of promising high-energy electrode material for Zn-ion batteries[58]. However, they also faced various issues. VOPO4·nH2O dissolves easily in aqueous solution, leading to poor cycling performance[61]. Concentrated ZnCl2 electrolyte was reported to prevent the dissolution of VOPO4·2H2O and protect Zn metal from hydrogen evolution reactions and dendrites[162,163]. Additionally, 29 M ZnCl2 was adopted to inhibit H+ cointercalation and dissolution of LiV2(PO4)3[162]. The addition of 70% PEG favored reducing free water in the electrolyte and improving the coulomb efficiency[164]. The presence of high concentration of oxygen vacancies largely improved Zn2+ diffusion kinetics in VOPO4·2H2O nanosheets [Figure 11A]. Mott-Schottky (impedance potential) measurements also showed that the electronic conductivity was greatly improved due to high concentration of O vacancies, which increases the carrier concentration by about
Figure 11. Kinetics in Zn//bulk-VOPO4 and Zn//bilayer-VOPO4 batteries. (A) GITT and (B) Mott-Schottky plots. Reproduced with permission[61]. Copyright 2021, Wiley-VCH GmbH. Material preparation and performance of Na3V2(PO4)2F3@rGO. (C) Synthesis procedure, (D) galvanostatic charge-discharge profiles at 0.5 C, and (E) Cycling performance at 15 C. Reproduced with permission[60]. Copyright 2023, Wiley-VCH GmbH.
(2) Energy storage mechanism
Safe and cost-effective aqueous Zn batteries are well-suited for large-scale applications. However, some reaction mechanisms of the cathodes are currently controversial. The dominant mechanisms include reversible intercalation of Zn2+/H+ or solvated Zn2+/H2O. Reversible or irreversible phase transitions accompany the ion intercalation process. Various by-products are also generated in the process, such as
Coinsertion of Zn2+/H+ resulted in variation of pH in the electrolyte, contributing to the formation of those by-products, which indirectly proved the insertion/extraction of H+. For example, ζ-V2O5 generated from the Cu0.34V2O5 cathode after charging to 1.3 V, suffered cointercalation of Zn2+ and H+ accompanying the formation of (Zn (OH)2)2(ZnSO4) (H2O)n [Figure 12A][152]. Reversible H+ insertion/extraction happened in various cathodes, such as Cu0.18V2O5·0.72H2O[169], Mn-modified V6O13[170], Zn0.36V2O5·nH2O[171], and etc., implied by appearance and disappearance of Zn4SO4(OH)6·4H2O, Zn2V3O7(OH)2·2H2O, or
Figure 12. (A) Zn-storage mechanism in Cu0.34V2O5. Reproduced with permission[152]. Copyright 2021, American Chemical Society. (B) Zn-storage mechanism in Mg0.34V2O5·0.84H2O. Reproduced with permission[45]. Copyright 2018, American Chemical Society. (C) Reaction mechanism of Cu3(OH)2V2O7·2H2O at 0.1 A g-1. Reproduced with permission[173]. Copyright 2019, Royal Society of Chemistry. (D) Zn-storage in MgV2O4. Reproduced with permission[174]. Copyright 2020, American Chemical Society.
In hydrated ions, solvation water reduces the effective charge density and increases the distance among neighboring cations, leading to decreased coulomb interactions, which is responsible for the high diffusion coefficient. For example, an interlayer spacing of ~13.2 Å was observed when the hydrated Zn ion was intercalated into a porous Mg0.34V2O5·0.84H2O cathode, which was much larger than the size of Zn2+ (~0.7 Å) [Figure 12B][45]. CaV4O9 exhibited an enhanced charge transfer process due to the cointercalation of Zn2+ and H2O[172]. Similarly, the content of interlayer water in the Zn0.25V2O5·nH2O cathode changed with the content of intercalated Zn[38].
Differently, intercalation of desolvated Zn2+ also happened in some circumstances. It was reported that the transformation from Cu2+ to metallic Cu0 particles occurred when desolvated Zn2+ intercalated into copper vanadates, e.g., from Cu3(OH)2V2O7·2H2O to Zn0.25V2O5·H2O; the processes were verified reversible after Zn extraction [Figure 12C][173]. Moreover, metallic Cu facilitates good electronic conductivity and superior rate capability. In another example, MgV2O4 with intercalated Zn formed in the discharge suffered from the extraction of both Zn2+ and Mg2+ in the charge process. Compared to Zn2+, Mg2+ was preferentially extracted. The resultant ZnxMgV2O4 [Figure 12D] served as a stable host for reversible Zn-storage, subsequently[174].
Supercapacitors
Compared with batteries, supercapacitors have lower sensitivity to temperature, better tolerance to charge/discharge cycles, superior power performance, and good cycling stability[175]. V-based materials are also considered as promising high-energy electrodes for electrochemical capacitors due to their excellent specific capacitance, long cycling stability, and good electrochemical reversibility[176], but poor electrical conductivity has hindered their further use in supercapacitors. A VOSO4 additive was reported to dramatically improve the cycling stability of V2O5-based supercapacitors, leading to 91.23% retention at 10 A g-1 after
CONCLUSIONS AND FUTURE PROSPECTS
This review combed recent advances of multivalent-ion storage applications for a variety of advanced V-based materials, including vanadium oxides, vanadates, vanadium sulfides, and V-based MXenes and phosphates. The features for typical structures were analyzed with representative materials. The relevant electrochemical properties and energy storage mechanisms for different advanced V-based electrodes were systemically discussed. The discussion covered devices of not only non-aqueous batteries and aqueous batteries but also supercapacitors. For different devices, challenges from poor conductivity, slow ion diffusion, dissolution and structural collapse, low operating voltage, etc. were discussed with the corresponding representative electrodes.
Based on the review, we disclosed that issues for V-based materials could be alleviated, to some extent, by common material engineering strategies such as nanosizing, doping, encapsulating, constructing vacancies and heterostructures, etc. Further, electrolyte design, e.g., highly concentrated electrolytes, organic/aqueous hybrid electrolytes, hybrid ions electrolytes, etc., are also beneficial to improve main factors of structure stability, ion storage capability and diffusion kinetics due to optimized surface/interface, weakened coulomb interactions, and enhanced storage pathways. Overall, to obtain better multivalent-ion storage applications for V-based materials, cooperation from material engineering and electrolyte design is possibly a promising avenue. Meanwhile, various advanced in-situ characterization techniques are also needed to clarify the relevant complex interactions between materials and electrolytes.
DECLARATIONS
Authors’ contributions
Proposed the topic of this review: Song H, Wang C
Prepared the manuscript: Guo W
Writing - review & editing: Guo W, Fu D, Song H, Wang C
Availability of data and materials
Not applicable.
Financial support and sponsorship
The work is financially supported by the National Natural Science Foundation of China (grant nos. 91963210, 52322107) and the Natural Science Foundation of Guangdong Province (grant nos. 2020B0101690001, 2022A1515010723).
Conflict of interest
All authors declared that there are no conflicts of interest.
Ethical approval and consent to participate
Not applicable.
Consent for publication
Not applicable.
Copyright
© The Author(s) 2024.
REFERENCES
1. Liu J, Bao Z, Cui Y, et al. Pathways for practical high-energy long-cycling lithium metal batteries. Nat Energy 2019;4:180-6.
2. Ren B, Cui H, Wang C. Self-supported graphene nanosheet-based composites as binder-free electrodes for advanced electrochemical energy conversion and storage. Electrochem Energy Rev 2022;5:2-27.
4. Wu L, Sun R, Xiong F, et al. A rechargeable aluminum-ion battery based on a VS2 nanosheet cathode. Phys Chem Chem Phys 2018;20:22563-8.
5. Canepa P, Sai Gautam G, Hannah DC, et al. Odyssey of multivalent cathode materials: open questions and future challenges. Chem Rev 2017;117:4287-341.
6. Song H, Li Y, Tian F, Wang C. Electrolyte optimization and interphase regulation for significantly enhanced storage capability in Ca-metal batteries. Adv Funct Mater 2022;32:2200004.
7. Song H, Su J, Wang C. Hybrid solid electrolyte interphases enabled ultralong life Ca-metal batteries working at room temperature. Adv Mater 2021;33:e2006141.
8. Song H, Wang C. Current status and challenges of calcium metal batteries. Adv Energy Sustain Res 2022;3:2100192.
9. Muldoon J, Bucur CB, Gregory T. Quest for nonaqueous multivalent secondary batteries: magnesium and beyond. Chem Rev 2014;114:11683-720.
10. Tang B, Shan L, Liang S, Zhou J. Issues and opportunities facing aqueous zinc-ion batteries. Energy Environ Sci 2019;12:3288-304.
11. Yan Y, Li B, Guo W, Pang H, Xue H. Vanadium based materials as electrode materials for high performance supercapacitors. J Power Sources 2016;329:148-69.
12. Xu X, Xiong F, Meng J, et al. Vanadium-based nanomaterials: a promising family for emerging metal-ion batteries. Adv Funct Mater 2020;30:1904398.
13. Liu S, Kang L, Kim JM, Chun YT, Zhang J, Jun SC. Recent advances in vanadium-based aqueous rechargeable zinc-ion batteries. Adv Energy Mater 2020;10:2000477.
14. Prasadam V, Bahlawane N, Mattelaer F, et al. Atomic layer deposition of vanadium oxides: process and application review. Mater Today Chem 2019;12:396-423.
15. Wan F, Niu Z. Design strategies for vanadium-based aqueous zinc-ion batteries. Angew Chem Int Ed 2019;58:16358-67.
16. Xu Y, Deng X, Li Q, et al. Vanadium oxide pillared by interlayer Mg2+ ions and water as ultralong-life cathodes for magnesium-ion batteries. Chem 2019;5:1194-209.
17. Miao X, Chen Z, Wang N, et al. Electrospun V2MoO8 as a cathode material for rechargeable batteries with Mg metal anode. Nano Energy 2017;34:26-35.
18. Hu P, Hu P, Vu TD, et al. Vanadium oxide: phase diagrams, structures, synthesis, and applications. Chem Rev 2023;123:4353-415.
19. Zou C, Fan L, Chen R, et al. Thermally driven V2O5 nanocrystal formation and the temperature-dependent electronic structure study. CrystEngComm 2012;14:626-31.
20. Huie MM, Bock DC, Takeuchi ES, Marschilok AC, Takeuchi KJ. Cathode materials for magnesium and magnesium-ion based batteries. Coord Chem Rev 2015;287:15-27.
21. Lee S, Ivanov IN, Keum JK, Lee HN. Epitaxial stabilization and phase instability of VO2 polymorphs. Sci Rep 2016;6:19621.
22. Wei M, Sugihara H, Honma I, Ichihara M, Zhou H. A New metastable phase of crystallized V2O4·0.25H2O nanowires: synthesis and electrochemical measurements. Adv Mater 2005;17:2964-9.
23. Chernova NA, Roppolo M, Dillon AC, Whittingham MS. Layered vanadium and molybdenum oxides: batteries and electrochromics. J Mater Chem 2009;19:2526.
24. Liu M, Su B, Tang Y, Jiang X, Yu A. Recent advances in nanostructured vanadium oxides and composites for energy conversion. Adv Energy Mater 2017;7:1700885.
25. Jin T, Li H, Li Y, Jiao L, Chen J. Intercalation pseudocapacitance in flexible and self-standing V2O3 porous nanofibers for high-rate and ultra-stable K ion storage. Nano Energy 2018;50:462-7.
26. Yi T, Qiu L, Qu J, Liu H, Zhang J, Zhu Y. Towards high-performance cathodes: design and energy storage mechanism of vanadium oxides-based materials for aqueous Zn-ion batteries. Coord Chem Rev 2021;446:214124.
27. Li H, He P, Wang Y, Hosono E, Zhou H. High-surface vanadium oxides with large capacities for lithium-ion batteries: from hydrated aerogel to nanocrystalline VO2(B), V6O13 and V2O5. J Mater Chem 2011;21:10999.
28. Shin J, Choi DS, Lee HJ, Jung Y, Choi JW. Hydrated Intercalation for high-performance aqueous zinc ion batteries. Adv Energy Mater 2019;9:1900083.
29. Zhang Y, Liu X, Xie G, et al. Hydrothermal synthesis, characterization, formation mechanism and electrochemical property of
30. Lv T, Peng Y, Zhang G, et al. How about vanadium-based compounds as cathode materials for aqueous zinc ion batteries? Adv Sci 2023;10:e2206907.
31. Liu Y, Xu L, Guo X, Lv T, Pang H. Vanadium sulfide based materials: synthesis, energy storage and conversion. J Mater Chem A 2020;8:20781-802.
32. Rout CS, Kim BH, Xu X, et al. Synthesis and characterization of patronite form of vanadium sulfide on graphitic layer. J Am Chem Soc 2013;135:8720-5.
33. Feng J, Sun X, Wu C, et al. Metallic few-layered VS2 ultrathin nanosheets: high two-dimensional conductivity for in-plane supercapacitors. J Am Chem Soc 2011;133:17832-8.
34. Yao K, Wu M, Chen D, et al. Vanadium tetrasulfide for next-generation rechargeable batteries: advances and challenges. Chem Rec 2022;22:e202200117.
35. Hu Z, Liu Q, Chou SL, Dou SX. Advances and challenges in metal sulfides/selenides for next-generation rechargeable sodium-ion batteries. Adv Mater 2017;29:1700606.
36. Sun R, Wei Q, Li Q, et al. Vanadium sulfide on reduced graphene oxide layer as a promising anode for sodium ion battery. ACS Appl Mater Interfaces 2015;7:20902-8.
37. Cheng S, Yao K, Zheng K, et al. Self-assembled VS4 hierarchitectures with enhanced capacity and stability for sodium storage. Energy Environ Mater 2022;5:592-8.
38. Kundu D, Adams BD, Duffort V, Vajargah SH, Nazar LF. A high-capacity and long-life aqueous rechargeable zinc battery using a metal oxide intercalation cathode. Nat Energy 2016;1:16119.
39. Yang Y, Tang Y, Liang S, et al. Transition metal ion-preintercalated V2O5 as high-performance aqueous zinc-ion battery cathode with broad temperature adaptability. Nano Energy 2019;61:617-25.
40. Sarkar S, Banda H, Mitra S. High capacity lithium-ion battery cathode using LiV3O8 nanorods. Electrochim Acta 2013;99:242-52.
41. Oka Y, Yao T, Yamamoto N. Hydrothermal synthesis and structure refinements of alkali-metal trivanadates AV3O8 (A = K, Rb, Cs). Mater Res Bull 1997;32:1201-9.
42. Krachodnok S, Haller KJ, Willaims ID. Improved synthesis of alkali metal vanadates using a hydrothermal method. Eng J 2012;16:19-28.
43. Wadsley AD. Crystal chemistry of non-stoichiometric pentavalent vandadium oxides: crystal structure of Li1+xV3O8. Acta Cryst 1957;10:261-7.
44. Peng S, Li L, Hu Y, et al. Fabrication of spinel one-dimensional architectures by single-spinneret electrospinning for energy storage applications. ACS Nano 2015;9:1945-54.
45. Ming F, Liang H, Lei Y, Kandambeth S, Eddaoudi M, Alshareef HN. Layered MgxV2O5·nH2O as cathode material for high-performance aqueous zinc ion batteries. ACS Energy Lett 2018;3:2602-9.
46. Wang J, Wang J, Jiang Y, et al. CaV6O16·2.8H2O with Ca2+ pillar and water lubrication as a high-rate and long-life cathode material for ca-ion batteries. Adv Funct Mater 2022;32:2113030.
47. Li J, Mccoll K, Lu X, et al. Multi-scale investigations of δ-Ni0.25V2O5·nH2O cathode materials in aqueous zinc-ion batteries. Adv Energy Mater 2020;10:2000058.
48. Liu Y, Li C, Xu J, et al. Electroactivation-induced spinel ZnV2O4 as a high-performance cathode material for aqueous zinc-ion battery. Nano Energy 2020;67:104211.
49. Tang C, Xiong F, Lan B, et al. Constructing a disorder/order structure for enhanced magnesium storage. Chem Eng J 2020;382:123049.
50. Ma XF, Li HY, Zhu X, et al. Switchable and strain-releasable Mg-ion diffusion nanohighway enables high-capacity and long-life pyrovanadate cathode. Small 2022;18:e2202250.
51. Liu Y, Li Q, Ma K, Yang G, Wang C. Graphene oxide wrapped CuV2O6 nanobelts as high-capacity and long-life cathode materials of aqueous zinc-ion batteries. ACS Nano 2019;13:12081-9.
52. Zhu K, Jiang W, Wang Z, et al. Hewettite ZnV6O16 · 8H2O with remarkably stable layers and ultralarge interlayer spacing for high-performance aqueous Zn-ion batteries. Angew Chem Int Ed 2023;62:e202213368.
53. Yang W, Yang W, Huang Y, Xu C, Dong L, Peng X. Reversible aqueous zinc-ion battery based on ferric vanadate cathode. Chin Chem Lett 2022;33:4628-34.
54. Wei T, Li Q, Yang G, Wang C. Highly reversible and long-life cycling aqueous zinc-ion battery based on ultrathin
55. Vo TN, Kim H, Hur J, Choi W, Kim IT. Surfactant-assisted ammonium vanadium oxide as a superior cathode for calcium-ion batteries. J Mater Chem A 2018;6:22645-54.
56. Wei T, Liu Y, Yang G, Wang C. Aluminum vanadate hollow spheres as zero-strain cathode material for highly reversible and durable aqueous zinc-ion batteries. Energy Storage Mater 2020;30:130-7.
57. Li Y, Liu Y, Chen J, et al. Polyaniline intercalation induced great enhancement of electrochemical properties in ammonium vanadate nanosheets as an advanced cathode for high-performance aqueous zinc-ion batteries. Chem Eng J 2022;448:137681.
58. Liu C, Massé R, Nan X, Cao G. A promising cathode for Li-ion batteries: Li3V2(PO4)3. Energy Storage Mater 2016;4:15-58.
59. Jian Z, Hu YS, Ji X, Chen W. NASICON-structured materials for energy storage. Adv Mater 2017;29:1601925.
60. Guan J, Huang Q, Shao L, et al. Polyanion-type Na3V2(PO4)2F3@rGO with high-voltage and ultralong-life for aqueous zinc ion batteries. Small 2023;19:e2207148.
61. Wu Z, Lu C, Ye F, et al. Bilayered VOPO4·2H2O nanosheets with high-concentration oxygen vacancies for high-performance aqueous zinc-ion batteries. Adv Funct Mater 2021;31:2106816.
62. Zheng J, Xu T, Xia G, Cui WG, Yang Y, Yu X. Water-stabilized vanadyl phosphate monohydrate ultrathin nanosheets toward high voltage Al-ion batteries. Small 2023;19:e2207619.
63. VahidMohammadi A, Hadjikhani A, Shahbazmohamadi S, Beidaghi M. Two-dimensional vanadium carbide (MXene) as a high-capacity cathode material for rechargeable aluminum batteries. ACS Nano 2017;11:11135-44.
65. Qureshi A, Abdelhay AH, Zaidi SA, et al. Emerging trends in niobium, vanadium, and molybdenum based MXenes applications. Crit Rev Solid State Mater Sci 2024;49:141-62.
66. Liu Y, Jiang Y, Hu Z, et al. In-situ electrochemically activated surface vanadium valence in V2C MXene to achieve high capacity and superior rate performance for Zn-ion batteries. Adv Funct Mater 2021;31:2008033.
67. Guan J, Shao L, Yu L, et al. Two-dimensional Mg0.2V2O5·nH2O nanobelts derived from V4C3 MXenes for highly stable aqueous zinc ion batteries. Chem Eng J 2022;443:136502.
68. Zhu J, Zhang X, Gao H, et al. VS4 anchored on Ti3C2 MXene as a high-performance cathode material for magnesium ion battery. J Power Sources 2022;518:230731.
69. Aurbach D, Lu Z, Schechter A, et al. Prototype systems for rechargeable magnesium batteries. Nature 2000;407:724-7.
70. An Q, Li Y, Deog Yoo H, et al. Graphene decorated vanadium oxide nanowire aerogel for long-cycle-life magnesium battery cathodes. Nano Energy 2015;18:265-72.
71. Wang J, Tan S, Zhang G, et al. Fast and stable Mg2+ intercalation in a high voltage NaV2O2(PO4)2F/rGO cathode material for magnesium-ion batteries. Sci China Mater 2020;63:1651-62.
72. Dong H, Liang Y, Tutusaus O, et al. Directing Mg-storage chemistry in organic polymers toward high-energy Mg batteries. Joule 2019;3:782-93.
73. Zhao Y, Wang D, Yang D, et al. Superior Mg2+ storage properties of VS2 nanosheets by using an APC-PP14Cl/THF electrolyte. Energy Storage Mater 2019;23:749-56.
74. Li Z, Ding S, Yin J, Zhang M, Sun C, Meng A. Morphology-dependent electrochemical performance of VS4 for rechargeable magnesium battery and its magnesiation/demagnesiation mechanism. J Power Sources 2020;451:227815.
75. Wang Y, Liu Z, Wang C, et al. Highly branched VS4 Nanodendrites with 1D atomic-Chain structure as a promising cathode material for long-cycling magnesium batteries. Adv Mater 2018;30:e1802563.
76. Ding S, Dai X, Tian Y, et al. Synergy strategy of electrical conductivity enhancement and vacancy introduction for improving the performance of VS4 magnesium-ion battery cathode. ACS Appl Mater Interfaces 2021;13:54005-17.
77. Pei C, Yin Y, Sun R, et al. Interchain-expanded vanadium tetrasulfide with fast kinetics for rechargeable magnesium batteries. ACS Appl Mater Interfaces 2019;11:31954-61.
78. Xue X, Chen R, Yan C, et al. One-step synthesis of 2-ethylhexylamine pillared vanadium disulfide nanoflowers with ultralarge interlayer spacing for high-performance magnesium storage. Adv Energy Mater 2019;9:1900145.
79. Ding S, Dai X, Li Z, et al. PVP-induced synergistic engineering of interlayer, self-doping, active surface and vacancies in VS4 for enhancing magnesium ions storage and durability. Energy Storage Mater 2022;47:211-22.
80. Mukherjee A, Taragin S, Aviv H, Perelshtein I, Noked M. Rationally designed vanadium pentoxide as high capacity insertion material for Mg-ion. Adv Funct Mater 2020;30:2003518.
81. Zuo C, Xiao Y, Pan X, et al. Organic-inorganic superlattices of vanadium oxide@polyaniline for high-performance magnesium-ion batteries. ChemSusChem 2021;14:2093-9.
82. Joe YS, Kang MS, Jang G, et al. Intercalation of bilayered V2O5 by electronically coupled PEDOT for greatly improved kinetic performance of magnesium ion battery cathodes. Chem Eng J 2023;460:141706.
83. Wu D, Zhuang Y, Wang F, Yang Y, Zeng J, Zhao J. High-rate performance magnesium batteries achieved by direct growth of honeycomb-like V2O5 electrodes with rich oxygen vacancies. Nano Res 2023;16:4880-7.
84. Tang H, Xiong F, Jiang Y, et al. Alkali ions pre-intercalated layered vanadium oxide nanowires for stable magnesium ions storage. Nano Energy 2019;58:347-54.
85. Rashad M, Zhang H, Asif M, Feng K, Li X, Zhang H. Low-cost room-temperature synthesis of NaV3O8·1.69H2O nanobelts for Mg batteries. ACS Appl Mater Interfaces 2018;10:4757-66.
86. Wang X, Zhang X, Zhao G, et al. Ether-water hybrid electrolyte contributing to excellent Mg ion storage in layered sodium vanadate. ACS Nano 2022;16:6093-102.
87. Tang B, Fang G, Zhou J, et al. Potassium vanadates with stable structure and fast ion diffusion channel as cathode for rechargeable aqueous zinc-ion batteries. Nano Energy 2018;51:579-87.
88. Li C, Wu W, Liu Y, et al. Facilitating Mg2+ diffusion in high potential LixV2(PO4)3 cathode material with a co-insertion strategy for rechargeable Mg-ion batteries. J Power Sources 2022;520:230853.
89. Zhang X, Xu X, Song B, et al. Towards a stable layered vanadium oxide cathode for high-capacity calcium batteries. Small 2022;18:e2107174.
90. Jeon B, Kwak HH, Hong S. Bilayered Ca0.28V2O5·H2O: high-capacity cathode material for rechargeable Ca-ion batteries and its charge storage mechanism. Chem Mater 2022;34:1491-8.
91. Purbarani ME, Hyoung J, Hong S. Crystal-water-free potassium vanadium bronze (K0.5V2O5) as a cathode material for Ca-ion batteries. ACS Appl Energy Mater 2021;4:7487-91.
92. Adil M, Sarkar A, Sau S, Muthuraj D, Mitra S. Non-aqueous rechargeable calcium-ion batteries based on high voltage zirconium-doped ammonium vanadium oxide cathode. J Power Sources 2022;541:231669.
93. Wang Y, Cai J, Han T, et al. In-situ growing polyaniline nano-spine array on FeVO4 nanobelts as high-performance rechargeable aluminum-ion battery cathode. Appl Surf Sci 2022;591:153157.
94. Singh S, Bairagi PK, Verma N. Candle soot-derived carbon nanoparticles: an inexpensive and efficient electrode for microbial fuel cells. Electrochim Acta 2018;264:119-27.
96. Ju S, Ye J, Meng Y, Xia G, Yu X. Pre-lithiated Li2V6O13 cathode enables high-energy aluminum-ion battery. Adv Energy Mater 2022;12:2201653.
97. Xing L, Owusu KA, Liu X, et al. Insights into the storage mechanism of VS4 nanowire clusters in aluminum-ion battery. Nano Energy 2021;79:105384.
98. Han X, Wu F, Zhao R, Bai Y, Wu C. Tremella-like vanadium tetrasulfide as a high-performance cathode material for rechargeable aluminum batteries. ACS Appl Mater Interfaces 2023;15:6888-901.
99. Li Q, Rui X, Chen D, et al. A high-capacity ammonium vanadate cathode for zinc-ion battery. Nanomicro Lett 2020;12:67.
100. Tan H, Chen D, Liu W, et al. Free-standing hydrated sodium vanadate papers for high-stability zinc-ion batteries. Batteries Supercaps 2020;3:254-60.
101. Mei Y, Liu Y, Xu W, Zhang M, Dong Y, Qiu J. Suppressing vanadium dissolution in 2D V2O5/MXene heterostructures via organic/aqueous hybrid electrolyte for stable zinc ion batteries. Chem Eng J 2023;452:139574.
102. Yagi S, Ichitsubo T, Shirai Y, et al. A concept of dual-salt polyvalent-metal storage battery. J Mater Chem A 2014;2:1144-9.
103. Sun R, Pei C, Sheng J, et al. High-rate and long-life VS2 cathodes for hybrid magnesium-based battery. Energy Storage Mater 2018;12:61-8.
104. Pei C, Xiong F, Sheng J, et al. VO2 nanoflakes as the cathode material of hybrid magnesium-lithium-ion batteries with high energy density. ACS Appl Mater Interfaces 2017;9:17060-6.
105. Hu X, Peng J, Xu F, Ding M. Rechargeable Mg2+/Li+, Mg2+/Na+, and Mg2+/K+ hybrid batteries based on layered VS2. ACS Appl Mater Interfaces 2021;13:57252-63.
106. Rashad M, Li X, Zhang H. Magnesium/lithium-ion hybrid battery with high reversibility by employing NaV3O8·1.69H2O nanobelts as a positive electrode. ACS Appl Mater Interfaces 2018;10:21313-20.
107. Zhao S, Li C, Zhang X, et al. An advanced Ca/Zn hybrid battery enabled by the dendrite-free zinc anode and a reversible calcification/decalcification NASICON cathode. Sci Bull 2023;68:56-64.
108. Liang Z, Tian F, Yang G, Wang C. Enabling long-cycling aqueous sodium-ion batteries via Mn dissolution inhibition using sodium ferrocyanide electrolyte additive. Nat Commun 2023;14:3591.
109. Zhang H, Cao D, Bai X. High rate performance of aqueous magnesium-ion batteries based on the δ-MnO2@carbon molecular sieves composite as the cathode and nanowire VO2 as the anode. J Power Sources 2019;444:227299.
110. Zhao Y, Chen Z, Mo F, et al. Aqueous rechargeable metal-ion batteries working at subzero temperatures. Adv Sci 2020;8:2002590.
111. Yang G, Xu X, Qu G, et al. An aqueous magnesium-ion battery working at -50 °C enabled by modulating electrolyte structure. Chem Eng J 2023;455:140806.
112. Zhang H, Ye K, Zhu K, et al. High-energy-density aqueous magnesium-ion battery based on a carbon-coated FeVO4 anode and a
113. Liu L, Wu YC, Rozier P, Taberna PL, Simon P. Ultrafast synthesis of calcium vanadate for superior aqueous calcium-ion battery. Research 2019;2019:6585686.
114. Dong L, Xu R, Wang P, et al. Layered potassium vanadate K2V6O16 nanowires: a stable and high capacity cathode material for calcium-ion batteries. J Power Sources 2020;479:228793.
115. Soundharrajan V, Nithiananth S, Lee J, Kim JH, Hwang J, Kim J. LiV3O8 as an intercalation-type cathode for aqueous aluminum-ion batteries. J Mater Chem A 2022;10:18162-9.
116. Kumar S, Satish R, Verma V, et al. Investigating FeVO4 as a cathode material for aqueous aluminum-ion battery. J Power Sources 2019;426:151-61.
117. Pang Q, Yang S, Yu X, et al. Realizing reversible storage of trivalent aluminum ions using VOPO4·2H2O nanosheets as cathode material in aqueous aluminum metal batteries. J Alloys Compd 2021;885:161008.
118. Wang P, Chen Z, Wang H, et al. A high-performance flexible aqueous Al ion rechargeable battery with long cycle life. Energy Storage Mater 2020;25:426-35.
119. Yang Q, Qu X, Cui H, et al. Rechargeable aqueous Mn-metal battery enabled by inorganic-organic interfaces. Angew Chem Int Ed 2022;61:e202206471.
121. Bi S, Wang S, Yue F, Tie Z, Niu Z. A rechargeable aqueous manganese-ion battery based on intercalation chemistry. Nat Commun 2021;12:6991.
122. Liu Y, Lu X, Lai F, et al. Rechargeable aqueous Zn-based energy storage devices. Joule 2021;5:2845-903.
123. Guo J, Ming J, Lei Y, et al. Artificial solid electrolyte interphase for suppressing surface reactions and cathode dissolution in aqueous zinc ion batteries. ACS Energy Lett 2019;4:2776-81.
124. Zhang N, Cheng F, Liu J, et al. Rechargeable aqueous zinc-manganese dioxide batteries with high energy and power densities. Nat Commun 2017;8:405.
125. Zhang W, Dai Y, Chen R, et al. Highly reversible zinc metal anode in a dilute aqueous electrolyte enabled by a pH buffer additive. Angew Chem Int Ed 2023;62:e202212695.
126. He P, Yan M, Zhang G, et al. Layered VS2 nanosheet-based aqueous Zn ion battery cathode. Adv Energy Mater 2017;7:1601920.
127. Jiao T, Yang Q, Wu S, et al. Binder-free hierarchical VS2 electrodes for high-performance aqueous Zn ion batteries towards commercial level mass loading. J Mater Chem A 2019;7:16330-8.
128. Yu D, Wei Z, Zhang X, et al. Boosting Zn2+ and NH4+ storage in aqueous media via in-situ electrochemical induced VS2/VOx heterostructures. Adv Funct Mater 2021;31:2008743.
129. Liu J, Peng W, Li Y, Zhang F, Fan X. A VS2@N-doped carbon hybrid with strong interfacial interaction for high-performance rechargeable aqueous Zn-ion batteries. J Mater Chem C 2021;9:6308-15.
130. Gao S, Ju P, Liu Z, et al. Electrochemically induced phase transition in a nanoflower vanadium tetrasulfide cathode for high-performance zinc-ion batteries. J Energy Chem 2022;69:356-62.
131. Qin H, Yang Z, Chen L, Chen X, Wang L. A high-rate aqueous rechargeable zinc ion battery based on the VS4@rGO nanocomposite. J Mater Chem A 2018;6:23757-65.
132. Yoo G, Koo B, An G. Nano-sized split V2O5 with H2O-intercalated interfaces as a stable cathode for zinc ion batteries without an aging process. Chem Eng J 2022;434:134738.
133. Yan M, He P, Chen Y, et al. Water-Lubricated Intercalation in V2O5·nH2O for high-capacity and high-rate aqueous rechargeable zinc batteries. Adv Mater 2018;30:1703725.
134. Li Y, Huang Z, Kalambate PK, et al. V2O5 nanopaper as a cathode material with high capacity and long cycle life for rechargeable aqueous zinc-ion battery. Nano Energy 2019;60:752-9.
135. Wei T, Li Q, Yang G, Wang C. High-rate and durable aqueous zinc ion battery using dendritic V10O24·12H2O cathode material with large interlamellar spacing. Electrochim Acta 2018;287:60-7.
136. Yang G, Wei T, Wang C. Self-healing lamellar structure boosts highly stable zinc-storage property of bilayered vanadium oxides. ACS Appl Mater Interfaces 2018;10:35079-89.
137. Jiang H, Gong W, Zhang Y, et al. Quench-tailored Al-doped V2O5 nanomaterials for efficient aqueous zinc-ion batteries. J Energy Chem 2022;70:52-8.
138. Zhao Y, Han C, Yang J, et al. Stable alkali metal ion intercalation compounds as optimized metal oxide nanowire cathodes for lithium batteries. Nano Lett 2015;15:2180-5.
139. Wang X, Zhang Z, Huang M, Feng J, Xiong S, Xi B. In situ electrochemically activated vanadium oxide cathode for advanced aqueous Zn-ion batteries. Nano Lett 2022;22:119-27.
140. Zhu K, Wei S, Shou H, et al. Defect engineering on V2O3 cathode for long-cycling aqueous zinc metal batteries. Nat Commun 2021;12:6878.
141. Cao Z, Chu H, Zhang H, et al. An in situ electrochemical oxidation strategy for formation of nanogrid-shaped V3O7·H2O with enhanced zinc storage properties. J Mater Chem A 2019;7:25262-7.
142. Cao H, Zheng Z, Norby P, Xiao X, Mossin S. Electrochemically induced phase transition in V3O7·H2O nanobelts/reduced graphene oxide composites for aqueous zinc-ion batteries. Small 2021;17:2100558.
143. Ding Y, Peng Y, Chen S, et al. Hierarchical porous metallic V2O3@C for advanced aqueous zinc-ion batteries. ACS Appl Mater Interfaces 2019;11:44109-17.
144. He P, Liu J, Zhao X, Ding Z, Gao P, Fan L. A three-dimensional interconnected V6O13 nest with a V5+-rich state for ultrahigh Zn ion storage. J Mater Chem A 2020;8:10370-6.
145. Shi W, Yin B, Yang Y, et al. Unravelling V6O13 diffusion pathways via CO2 modification for high-performance zinc ion battery cathode. ACS Nano 2021;15:1273-81.
146. Chen L, Ruan Y, Zhang G, et al. Ultrastable and high-performance Zn/VO2 battery based on a reversible single-phase reaction. Chem Mater 2019;31:699-706.
147. Zhu K, Wu T, Huang K. A high-voltage activated high-erformance cathode for aqueous Zn-ion batteries. Energy Storage Mater 2021;38:473-81.
148. Wei T, Li Q, Yang G, Wang C. An electrochemically induced bilayered structure facilitates long-life zinc storage of vanadium dioxide. J Mater Chem A 2018;6:8006-12.
149. Tang Z, Zou R, Chen X, Li Z, Lei G. Solvothermal synthesis of VO2 and in situ electrochemical transformation of Zn2V2O7 as cathode for long-life aqueous zinc-ion batteries. J Power Sources 2023;569:233006.
150. Deng S, Li H, Chen B, et al. High performance of Mn-doped VO2 cathode for aqueous zinc-ion batteries: an insight into Zn2+ storage mechanism. Chem Eng J 2023;452:139115.
151. Ma L, Li N, Long C, et al. Achieving both high voltage and high capacity in aqueous zinc-ion battery for record high energy density. Adv Funct Mater 2019;29:1906142.
152. Chae MS, Attias R, Dlugatch B, Gofer Y, Aurbach D. Multifold electrochemical protons and zinc ion storage behavior in copper vanadate cathodes. ACS Appl Energy Mater 2021;4:10197-202.
153. Alfaruqi MH, Mathew V, Song J, et al. Electrochemical zinc intercalation in lithium vanadium oxide: a high-capacity zinc-ion battery cathode. Chem Mater 2017;29:1684-94.
154. Li Q, Liu Y, Ma K, Yang G, Wang C. In situ Ag nanoparticles reinforced pseudo-Zn-air reaction boosting Ag2V4O11 as high-performance cathode material for aqueous zinc-ion batteries. Small Methods 2019;3:1900637.
155. Wan F, Huang S, Cao H, Niu Z. Freestanding potassium vanadate/carbon nanotube films for ultralong-life aqueous zinc-ion batteries. ACS Nano 2020;14:6752-60.
156. Zhu K, Wu T, Huang K. NaCa0.6V6O16·3H2O as an ultra-stable cathode for Zn-ion batteries: the roles of pre-inserted dual-cations and structural water in V3O8 layer. Adv Energy Mater 2019;9:1901968.
157. Xia C, Guo J, Lei Y, Liang H, Zhao C, Alshareef HN. Rechargeable aqueous zinc-ion battery based on porous framework zinc pyrovanadate intercalation cathode. Adv Mater 2018;30:1705580.
158. Peng Z, Wei Q, Tan S, et al. Novel layered iron vanadate cathode for high-capacity aqueous rechargeable zinc batteries. Chem Commun 2018;54:4041-4.
159. Wang X, Xi B, Feng Z, et al. Layered (NH4)2V6O16·1.5H2O nanobelts as a high-performance cathode for aqueous zinc-ion batteries. J Mater Chem A 2019;7:19130-9.
160. Jiang Y, Wu Z, Ye F, et al. Spontaneous knitting behavior of 6.7-nm thin (NH4)0.38V2O5 nano- ribbons for binder-free zinc-ion batteries. Energy Storage Mater 2021;42:286-94.
161. Li S, Yu D, Liu J, et al. Quantitative regulation of interlayer space of NH4V4O10 for fast and durable Zn2+ and NH4+ storage. Adv Sci 2023;10:e2206836.
162. Shi HY, Song Y, Qin Z, et al. Inhibiting VOPO4·xH2O decomposition and dissolution in rechargeable aqueous zinc batteries to promote voltage and capacity stabilities. Angew Chem Int Ed 2019;58:16057-61.
163. Zhang W, Dong M, Jiang K, et al. Self-repairing interphase reconstructed in each cycle for highly reversible aqueous zinc batteries. Nat Commun 2022;13:5348.
164. Li C, Kingsbury R, Zhou L, Shyamsunder A, Persson KA, Nazar LF. Tuning the solvation structure in aqueous zinc batteries to maximize Zn-ion intercalation and optimize dendrite-free zinc plating. ACS Energy Lett 2022;7:533-40.
165. Hu L, Wu Z, Lu C, Ye F, Liu Q, Sun Z. Principles of interlayer-spacing regulation of layered vanadium phosphates for superior zinc-ion batteries. Energy Environ Sci 2021;14:4095-106.
166. Hu P, Zhu T, Wang X, et al. Aqueous Zn//Zn(CF3SO3)2//Na3V2(PO4)3 batteries with simultaneous Zn2+/Na+ intercalation/de-intercalation. Nano Energy 2019;58:492-8.
167. Pang Q, Sun C, Yu Y, et al. H2V3O8 nanowire/graphene electrodes for aqueous rechargeable zinc ion batteries with high rate capability and large capacity. Adv Energy Mater 2018;8:1800144.
168. Chen X, Kong Q, Wu X, et al. V2O3@C optimized by carbon regulation strategy for ultra long-life aqueous zinc-ion batteries. Chem Eng J 2023;451:138765.
169. Ren J, Hong P, Ran Y, Chen Y, Xiao X, Wang Y. Binder-free three-dimensional interconnected CuV2O5·nH2O nests as cathodes for high-loading aqueous zinc-ion batteries. Inorg Chem Front 2022;9:792-804.
170. Li X, Li M, Yang Q, et al. In situ electrochemical synthesis of MXenes without acid/alkali usage in/for an aqueous zinc ion battery. Adv Energy Mater 2020;10:2001791.
171. Zhang X, Xue F, Sun X, et al. High-capacity zinc vanadium oxides with long-term cyclability enabled by in-situ electrochemical oxidation as zinc-ion battery cathode. Chem Eng J 2022;445:136714.
172. Du Y, Wang X, Zhang Y, et al. High mass loading CaV4O9 microflowers with amorphous phase transformation as cathode for aqueous zinc-ion battery. Chem Eng J 2022;434:134642.
173. Shan L, Zhou J, Han M, et al. Reversible Zn-driven reduction displacement reaction in aqueous zinc-ion battery. J Mater Chem A 2019;7:7355-9.
174. Tang W, Lan B, Tang C, et al. Urchin-like spinel MgV2O4 as a cathode material for aqueous zinc-ion batteries. ACS Sustain Chem Eng 2020;8:3681-8.
175. Salanne M, Rotenberg B, Naoi K, et al. Efficient storage mechanisms for building better supercapacitors. Nat Energy 2016;1:16070.
176. Guo J, Li L, Luo J, et al. Polypyrrole-assisted nitrogen doping strategy to boost vanadium dioxide performance for wearable nonpolarity supercapacitor and aqueous zinc-ion battery. Adv Energy Mater 2022;12:2201481.
177. Lee Y, Yoo G, Jo Y, An H, Koo B, An G. Interfacial electrochemical media-engineered tunable vanadium zinc hydrate oxygen defect for enhancing the redox reaction of zinc-ion hybrid supercapacitors. Adv Energy Mater 2023;13:2300630.
178. Fu Q, Wu X, Luo X, et al. High-voltage aqueous Mg-ion batteries enabled by solvation structure reorganization. Adv Funct Mater 2022;32:2110674.
179. Wu D, Zeng J, Hua H, Wu J, Yang Y, Zhao J. NaV6O15: a promising cathode material for insertion/extraction of Mg2+ with excellent cycling performance. Nano Res 2020;13:335-43.
180. Tang H, Chao F, Chen H, et al. Water-lubricated aluminum vanadate for enhanced rechargeable magnesium ion storage. Small 2022;18:e2203525.
181. Deng X, Xu Y, An Q, et al. Manganese ion pre-intercalated hydrated vanadium oxide as a high-performance cathode for magnesium ion batteries. J Mater Chem A 2019;7:10644-50.
182. Xu X, Duan M, Yue Y, et al. Bilayered Mg0.25V2O5·H2O as a stable cathode for rechargeable Ca-ion batteries. ACS Energy Lett 2019;4:1328-35.
183. Chae MS, Setiawan D, Kim HJ, Hong ST. Layered iron vanadate as a high-capacity cathode material for nonaqueous calcium-ion batteries. Batteries 2021;7:54.
184. Gao W, Michalička J, Pumera M. Hierarchical atomic layer deposited V2O5 on 3D printed nanocarbon electrodes for high-performance aqueous zinc-ion batteries. Small 2022;18:e2105572.
185. Li Z, Ren Y, Mo L, et al. Impacts of oxygen vacancies on zinc ion intercalation in VO2. ACS Nano 2020;14:5581-9.
186. Chen H, Chen L, Meng J, et al. Synergistic effects in V3O7/V2O5 composite material for high capacity and long cycling life aqueous rechargeable zinc ion batteries. J Power Sources 2020;474:228569.
187. He D, Peng Y, Ding Y, et al. Suppressing the skeleton decomposition in Ti-doped NH4V4O10 for durable aqueous zinc ion battery. J Power Sources 2021;484:229284.
188. He P, Yan M, Liao X, Luo Y, Mai L, Nan C. Reversible V3+/V5+ double redox in lithium vanadium oxide cathode for zinc storage. Energy Storage Mater 2020;29:113-20.
189. He P, Zhang G, Liao X, et al. Sodium ion stabilized vanadium oxide nanowire cathode for high-performance zinc-ion batteries. Adv Energy Mater 2018;8:1702463.
190. Yi H, Zuo C, Ren H, et al. Structure evolution and energy storage mechanism of Zn3V3O8 spinel in aqueous zinc batteries. Nanoscale 2021;13:14408-16.
Cite This Article
Export citation file: BibTeX | RIS
OAE Style
Guo W, Fu D, Song H, Wang C. Advanced V-based materials for multivalent-ion storage applications. Energy Mater 2024;4:400026. http://dx.doi.org/10.20517/energymater.2023.82
AMA Style
Guo W, Fu D, Song H, Wang C. Advanced V-based materials for multivalent-ion storage applications. Energy Materials. 2024; 4(2): 400026. http://dx.doi.org/10.20517/energymater.2023.82
Chicago/Turabian Style
Guo, Weihua, Danchen Fu, Huawei Song, Chengxin Wang. 2024. "Advanced V-based materials for multivalent-ion storage applications" Energy Materials. 4, no.2: 400026. http://dx.doi.org/10.20517/energymater.2023.82
ACS Style
Guo, W.; Fu D.; Song H.; Wang C. Advanced V-based materials for multivalent-ion storage applications. Energy Mater. 2024, 4, 400026. http://dx.doi.org/10.20517/energymater.2023.82
About This Article
Copyright
Data & Comments
Data
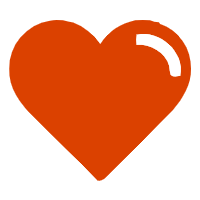

Comments
Comments must be written in English. Spam, offensive content, impersonation, and private information will not be permitted. If any comment is reported and identified as inappropriate content by OAE staff, the comment will be removed without notice. If you have any queries or need any help, please contact us at support@oaepublish.com.