Beyond energy harvesting: a review on the critical role of MXene in triboelectric nanogenerator
Abstract
In the field of advanced materials and energy harvesting, MXene has played a pivotal role in advancing the development of triboelectric nanogenerators (TENGs). This contribution is notable not only in terms of enhancing the performance of TENGs but also in expanding their application range. A comprehensive review of MXene materials is offered herein to delve into the significant impact of MXene on the growing efficiency of energy harvesting and widening application in areas ranging from energy harvesters to physiochemical sensors to self-powered intelligent systems. We begin with the fundamentals of MXene and TENGs, then highlight how MXene improves TENGs via its integration into the triboelectrification and electrode layers to increase the electronegativity, charge density, and introduce self-healing and stretchability. The discussion then extends to the modifications in MXene that boost the electrical output, stability, and collection efficiency of TENGs. Additionally, the review covers the diverse applications of MXene-based TENGs in extreme environments, respiratory monitoring, and multi-purpose devices, emphasizing its critical role in promoting TENGs to future self-powered intelligent systems.
Keywords
INTRODUCTION
MXene materials, a class of two-dimensional (2D) transition metal carbides and nitrides, are redefining the landscape of material science with their extraordinary properties. They are characterized by their metallic conductivity, high tensile strength, and excellent electromechanical properties[1]. Originating from the family of MAX phases, MXenes are produced by etching out the A-layer from the MAX phase, resulting in a structure that is both flexible and strong. Their surface, rich in functional groups such as -OH, -F, and -O, contributes to their unique chemical and physical properties[2]. This makes them ideal for a multitude of applications, including but not limited to energy storage, catalysis, electromagnetic shielding, and even biomedical applications[3]. Their versatility is further amplified by their ability to form composites with polymers and other materials, enhancing their applicability in various innovative technologies[4].
MXene-based triboelectric nanogenerators (TENGs) distinguish themselves through their superior electrical conductivity, mechanical flexibility, and surface functional ability, traits that are pivotal for enhancing the triboelectric effect and, thus, the overall performance of TENGs[5]. Compared to other 2D materials such as graphene, hexagonal boron nitride (h-BN), and transition metal dichalcogenides (TMDs), MXenes offer a unique combination of metallic conductivity and hydrophilicity thanks to their rich surface chemistry[6]. This not only improves charge transfer efficiency but also allows for fine-tuning of surface properties to maximize energy harvesting from diverse environmental sources. While graphene and TMDs excel in mechanical strength and chemical stability, their relatively inert surfaces and lesser conductivity can limit their performance in TENG applications. Conversely, the combination of high conductivity, flexibility, and tunable surface chemistry in MXenes renders them particularly advantageous for robust and efficient energy harvesting under varying conditions[7].
MXenes, due to their high surface activity and presence of transition metals, are susceptible to oxidation when exposed to air and moisture. This oxidative degradation can significantly impair their electrical and mechanical properties, posing challenges for their long-term performance in TENGs and other applications[8,9]. The degradation mechanism of MXene materials primarily involves the reaction of surface functional groups (-OH, -F, and -O) with atmospheric oxygen and water vapor, leading to the formation of oxides and hydroxides[10]. This process not only affects the conductivity of MXenes but also their structural integrity. To mitigate these effects, several strategies have been proposed and are actively being researched. One effective approach is the encapsulation of MXene flakes within protective layers, such as polymers, graphene, and h-BN, which can shield MXenes from direct exposure to the atmosphere while maintaining their flexibility and conductivity. Another strategy involves storing and processing MXene materials in inert atmospheres or under vacuum conditions to prevent oxidation during fabrication and handling[11,12]. Additionally, chemical modification of MXene surfaces with more stable functional groups or the application of anti-oxidation coatings can further enhance their resistance to environmental degradation[13].
TENGs, on the other hand, are a novel energy-harvesting technology that leverages the ancient principle of the triboelectric effect, combined with modern nanotechnology[14-17]. At their core, they operate by converting mechanical energy into electrical energy through the contact and separation of two different materials. This principle is akin to the static electricity phenomenon but is harnessed in a much more sophisticated and controlled manner[18-20]. TENGs are distinguished by their low-cost, lightweight nature, and their ability to harvest energy from various sources such as human motion, wind, water waves, and more[21,22]. Their flexibility in design and operation modes makes them highly adaptable for integration into various environments and applications, from powering small electronic devices and sensors to being potential solutions for large-scale energy generation problems[23].
Despite the promising attributes of TENGs, they are not without limitations. One of the primary challenges lies in their material durability, especially under diverse environmental conditions. The efficiency of TENGs can be significantly affected by factors such as humidity, temperature fluctuations, and mechanical wear over time[24,25]. Additionally, the electrical output of TENGs, while impressive for small-scale applications, often requires enhancement to meet the demands of larger or more power-intensive applications[26-28]. These limitations have sparked a search for innovations in materials and design that can elevate the robustness, efficiency, and versatility of TENGs, ensuring their practicality and effectiveness in a wider range of applications. Incorporating MXene materials into TENG technology presents a transformative solution to these challenges. MXenes, with their exceptional conductive properties and mechanical strength, synergistically enhance the performance of TENGs[29-31] [Figure 1]. When integrated into TENG structures, MXenes can improve the durability and stability of the devices, ensuring consistent performance under various environmental conditions. This integration also results in an increased electrical output, broadening the scope of applications for TENGs[32].
In the realm of nanogenerators for energy harvesting, TENGs, piezoelectric nanogenerators (PENGs), and pyroelectric nanogenerators (PyNGs) each capitalize on distinct physical phenomena - triboelectric, piezoelectric, and pyroelectric effects, respectively - to convert ambient energy into electrical energy. While PENGs excel in converting mechanical stress into electricity and are highly efficient under specific conditions, their reliance on materials with intrinsic piezoelectric properties can be a limitation[26]. Additionally, PyNGs are adept at harvesting energy from temperature fluctuations, yet their efficiency and response rate are constrained by the narrow temperature ranges and slow thermal responses. TENGs emerge as a versatile and efficient alternative, capable of harvesting energy from a wide variety of mechanical motions, owing to their simple fabrication process, low-cost material requirements, and exceptional energy conversion efficiency. The integration of TENGs with MXene materials, which are known for their outstanding conductivity, mechanical strength, and flexibility, further enhances their performance, making TENGs especially promising for applications in wearable electronics, flexible sensors, and the Internet of Things (IoT)[33-35].
In this work, we provide a comprehensive review of the multifaceted role of MXene in enhancing the functionality and efficiency of TENGs. Our examination delves into the critical aspects of contributions of MXene to TENGs, ranging from the significant improvement in the electronegativity and charge density of their friction layers to the notable enhancement of the output, self-repair capabilities, and stretchability of the electrode layer. We explore various physical and chemical modification methods that further augment the frictional electrical output of TENGs based on MXene, emphasizing expanding collection channels, improving output, and increasing stability. Additionally, the review casts light on the expansive and diverse applications of MXene-based TENGs (M-TENG) in extreme environments, highlighting their high-impact resistance, durability, and unique self-healing and tensile properties. We also cover their groundbreaking application in respiratory detection, including ammonia (NH3) and formaldehyde sensing, along with their use in multifunctional devices for tactile sensing, hydrogen production, electrochemical cathodic protection, and as components of wearable heaters. This comprehensive analysis aims to underline the pivotal role of MXene in advancing TENG technology and its broad array of applications across various sectors.
THE PRINCIPLE OF M-TENG
Brief overview of MXene
The MXene material was initially created by selectively removing the A layer from the MAX phase. In this phase, “M” is an early transition metal, “A” represents a group IIIA or IVA element, and "X" indicates either carbon or nitrogen[36]. This process alters the structure of the MAX phase into 2D layers reminiscent of graphene, hence the moniker “MXene”. These materials are typically represented by the formula Mn+1XnTx (where n ranges from 1 to 4). The "T" in the formula denotes various functional groups such as -F, -OH, and -O, which coat the surface of MXene during the etching stage. To date, researchers have successfully synthesized over 30 distinct MXene variants.
The creation of MXene primarily involves a top-down approach, starting with the chemical exfoliation of the parent MAX phase[37,38]. The most widespread technique for MXene production involves etching with hydrofluoric acid (HF) or similar solutions (such as HCl combined with LiF), followed by introducing an intercalant (such as metal or organic cations) to assist in delamination. This approach is notable for its ability to generate MXenes with a high concentration of -F groups on their surfaces under relatively mild temperature conditions[39,40].
The unique aspect of MXenes is their ability to be synthesized with various compositions and surface functionalities, leading to a diverse range of electrical, mechanical, and chemical properties[41]. This versatility has made MXenes a material of interest for applications in energy storage, such as supercapacitors (SCs) and batteries, sensors, electronic devices, photothermal therapy, and environmental remediation.
The synthesis of MXenes typically involves the selective etching of the "A" layers from their parent MAX phases (where "A" represents elements such as Al, Si, etc.) using HF or other etchants. This process results in the exfoliation of the layered structure, yielding MXene sheets that are just a few atoms thick. These sheets exhibit high electrical conductivity, flexibility, and hydrophilicity, contributing to their suitability for various applications[42].
In addressing the degradation of MXene during the fabrication of TENG electrodes, it is crucial to recognize that exposure to the outer atmosphere leads to oxidation, a primary cause of material degradation. MXene, a class of 2D transition metal carbides, nitrides, and carbonitrides, is particularly susceptible to degradation when exposed to air, moisture, and ultraviolet (UV) light, which can severely affect its electrical conductivity and overall performance in electrode applications. To mitigate these effects, preservation strategies such as storing MXene under inert atmospheres (e.g., argon or nitrogen), applying protective coatings or surface modifications to shield against environmental factors, and incorporating antioxidants to neutralize reactive oxygen species are essential[43,44]. Additionally, adopting advanced fabrication techniques that minimize exposure to degradative conditions can further preserve the integrity of MXene. By understanding these degradation mechanisms and implementing effective preservation measures, the longevity and efficiency of M-TENG electrodes can be significantly enhanced, ensuring their viability for various applications.
The potential of MXenes in TENGs, wearable electronics, and various sensors is particularly noteworthy. They have shown remarkable performance in enhancing the triboelectric properties of TENGs and improving the sensitivity and selectivity of sensors. This has opened up new avenues in the field of self-powered devices and advanced sensing technologies, further underscoring the versatility and potential of MXenes as cutting-edge materials.
MXene enhances electron transport
The layered structure of MXenes supports the fast transport of electrons through the material, a critical factor in reducing the internal resistance of TENGs and thus increasing their overall efficiency. The presence of surface terminations and defects within the MXene layers further contributes to electron trapping, a mechanism that can significantly enhance the charge density on the surface of TENGs. This increased charge density directly translates to a higher output performance of the TENG, making MXenes an excellent material choice for the triboelectric layer or as an additive to other composite materials used in TENG construction[45].
Moreover, the versatility of MXenes in terms of tunable surface chemistry and interlayer spacing allows for the engineering of specific properties to match the requirements of different TENG applications. By optimizing the type and amount of surface functional groups, researchers can tailor the electron affinity and surface energy of MXenes, further improving their effectiveness in electron transport and trapping mechanisms[46]. This adaptability not only enhances the energy conversion efficiency but also opens up possibilities for developing TENGs responsive to various mechanical energy sources in the environment, from human motion to vibrational energy in machines.
Figure 2 presents a compelling visualization of the charge density difference in Ti3C2O0.24(OH)1.28F0.48 MXene, comparing ordered versus random distributions of surface terminations[46]. This figure crucially illustrates how the presence and arrangement of functional groups (O, OH, and F) on the MXene surface significantly influence the charge distribution of the material, a key factor in its electronic and surface chemistry properties.
Figure 2. Minimizing similarity metrics for the analysis of Charge Density Variations in Ti3C2O0.24(OH)1.28F0.48. The depicted cyan and yellow areas signify zones of reduced and enhanced charge, respectively. An insert illustrates a two-dimensional cross-section of the charge density variance as seen from the (100) orientation, setting the isovalue limits from -0.03 to +0.03 electrons per cubic angstrom. A specific area marked in red within the insert accentuates a locally generated dipole on the surface. (A) Ordered Termination Surface of Ti3C2O0.24(OH)1.28F0.48. (B) Random Termination Surfaces of Ti3C2O0.24(OH)1.28F0.48[46].
In both the ordered and random configurations, we observe charge depletion and accumulation areas, particularly around the surface adatoms. Charge accumulation is pronounced near the electronegative O and F sites, while a noticeable charge depletion is localized at the hydrogen atom of the hydroxyl (OH) groups. This differential charge distribution is indicative of the polar nature of the OH group, which significantly influences the overall charge density on the MXene surface.
In summary, incorporating MXenes into TENGs leverages their exceptional electrical conductivity, surface chemistry, and structural properties to enhance electron transport. This leads to a notable improvement in the energy harvesting efficiency of TENGs, making them more viable for a wide range of applications, from wearable electronics to self-powered sensors in smart grids.
Brief overview of TENG
TENGs primarily utilize the triboelectric effect coupled with electrostatic induction to transform biomechanical energy into electrical power, which is essential for operating various medical devices. The underlying principle involves electron transfer between two materials of differing electronegativities upon contact. As these materials separate, the electrostatic induction phenomenon prompts electron movement across an external circuit. Through continuous repetition of this mechanism, TENGs can generate an alternating current (AC) output. This core concept allows for classifying TENGs into four unique operation modes, as depicted in Figure 3A including the Vertical Contact-Separation (CS)[47,48], Lateral Sliding (LS)[49,50], Single-Electrode (SE)[51-54], and Freestanding Triboelectric-Layer (FT) Modes[21,55].
Figure 3. (A) Vertical contact-separation mode, Lateral sliding mode, Single-electrode mode, and Freestanding triboelectric-layer mode[15]. Zhao et al. (2020), John Wiley and Sons[15]. (B) Two atoms at a distance greater than the bonding length, illustrating initial attraction without contact. (C) Atoms within the repulsive force region, showing interaction potential with a distance shorter than the bonding length but not leading to electron transition. (D) Separated electron clouds of two atoms before contact, depicting the stage prior to electron transition. (E) Overlapped electron clouds under mechanical pressure, showing lowered potential barrier and enabling electron transition[58].
In the Vertical CS Mode, two materials with different electronegativities come into vertical contact, facilitating electron exchange at the interface. Upon separation, a potential difference arises between attached electrodes due to electrostatic induction, leading to current flow through a connecting wire. This potential diminishes as the materials reunite, only to be reestablished upon subsequent separations, thus producing an AC.
The LS Mode operates on a principle analogous to the Vertical CS Mode, with the primary distinction being the change in relative displacement from vertical to horizontal. Through continuous horizontal movements, an AC is generated.
The SE Mode is characterized by its straightforward approach, leveraging the Earth as an inherent electrode. It operates by establishing a potential difference between a metallic electrode and the Earth via electrostatic induction, thereby generating a current.
In the FT Mode, the technique involves situating a pre-charged object amidst two electrodes, each bonded to a dielectric layer. The transit of this charged entity across the electrodes modifies the potential difference, producing a current.
Wang et al. have recently identified electron transfer as the primary mechanism facilitating charge exchange (CE) in solid-solid interactions[56]. This process is effectively explained through the surface states and Fermi level models for dielectrics and metals, respectively. When the separation between two materials exceeds the bond length, the atoms are drawn to each other, as depicted in Figure 3B. However, CE is observed to occur only when the distance between atoms is less than the bond length, within the zone of repulsive force in their interaction potential, as illustrated in Figure 3C. An innovative model, named the overlapped electron cloud model, was introduced to account for electron transition in general scenarios. As shown in Figure 3D, the electron clouds of two distinct materials stay separate until they come into atomic-scale contact[57]. Upon closer proximity and eventual contact under stress, the electron clouds overlap significantly, reducing the potential barrier and enabling electron transition, as shown in Figure 3E[58]. This necessitates mechanical pressure to decrease the interatomic distance and enhance electron cloud overlap. Termed the Wang et al. transition for CE, this model offers a comprehensive framework for understanding CE across various materials and could be adapted for additional CE scenarios. Furthermore, photon emission during this process has been theorized, pending experimental confirmation[58].
From a theoretical standpoint, the versatility in material selection for the triboelectric layer and electrodes in TENGs allows the introduction of various functional materials, including MXenes, for promoting a wide range of applications, particularly in customizing devices according to individual patient requirements.
THE ROLE OF MXENE IN TENG
The introduction of MXene, a class of 2D transition metal carbides, nitrides, and carbonitrides, has significantly revolutionized TENG technology [Table 1]. In this roadmap, MXene stands out for its exceptional electrical conductivity, mechanical flexibility, and high surface area, making it an ideal material for enhancing the performance and versatility of TENGs. The unique properties of MXene contribute to increased charge generation, improved energy efficiency, and the development of more robust and versatile TENGs suitable for diverse applications.
The performance of M-TENG
Data | Material composition | Energy source | Output | Applications |
2019[59] | PDMS/MXene film, LIG electrode | Leaf swing, writing | Voltage: ~119 V, Current: ~11 μA, Power: ~609.1 mW/m2 | Energy harvesting from agriculture, human activities, robotics |
2021[60] | PDMS/Mxenes composite, AgNWs electrodes | Mechanical, light | Voc: 453 V, Isc: 131 μA | Sound instructions, wind speed detection |
2022[61] | Nanoporous cobalt oxide/silicone, MXene/silicone nanocomposite, Conductive fabric electrode | Biomechanical motion | Power density: 10.4 W/m2 | Self-powered biomotion sensors, foot pressure sensors, wearable keyboards |
2022[62] | Ag flake electrodes, PTFE, Ecoflex | Human motion, vibrations | Voltage: 65 V, Current: 15.5 μA, Power: 1.2 μW | Wearable electronics, self-powered sensors |
2023[63] | PDMS dielectric, graphene electrodes, freestanding mode | Mechanical energy (motion, vibrations) | Voltage: 236 V, Short-circuit current: 128 μA, Power density: 35.9 mW/m2 | Energy harvesting, self-powered wearable devices |
2023[64] | Porous PDMS dielectric, carbon cloth electrode | Mechanical energy (hand tapping, vibrations) | Voltage: 106 V, Current: 8.9 μA, Power density: 13.2 mW/m2 | Self-powered sensors, energy harvesting |
2021[65] | Electrospun PVDF-TrFE/MXene nanofiber mat | Human finger tapping | Power density: 4.02 W/m2 at 4 MΩ | Self-powered switches for smart home appliances |
2023[66] | MXene, cotton fabric electrode, liquid silicon rubber | Mechanical energy (press/release) | Voltage: ~400 V, Current: ~680 nA | Self-charging power systems, wearable electronics |
2021[67] | Porous PDMS sponge TENG, SF@MXene-A-PDMS | Mechanical energy (hand tapping, vibrations) | Power density: 13.25 W/m2, Voltage: 2.3× previous best | Energy harvesting, sensitive self-powered sensing, wearable devices |
2020[68] | CNFs/MXene liquid electrode, silicone rubber capsule | Mechanical energy (human movements) | Voc: ~300 V, Isc: ~5.5 µA, Qsc: ~120 nC | Self-charging power systems, monitoring human movements, wearable electronics |
2022[69] | Silicone-incorporated nanoporous cobalt oxide, MXene/silicone nanocomposite, Conductive fabric electrodes | Biomechanical motion | Voc: 940 V, Current density: 65.5 mA/m2, Charge density: 550 µC/m2 | Self-powered sensors, wearable electronics |
2023[70] | Flexible MXene-based hydrogel electrode, Silicone rubber triboelectric layer | Mechanical energy | Voc: 95.04 V, Isc: 1.38 µA, Qsc: 30.84 nC | Energy harvesting, implantable storage devices, wearable health monitoring |
2021[71] | MXene/PVA hydrogel, Silicone rubber triboelectric layer | Mechanical energy | Voc: 230 V, Isc: Not specified, Qsc: Not specified | Wearable movement monitoring, stroke recognition, energy harvesting |
2020[72] | Mxene-AgNWs-Mxene-polyurethane (MAMP) electrode | Human movements | Voc: 38 V, Current density: 1.67 mA/m2, Power density: 7.22 mW/m2 | Energy harvester, motion sensor, self-powered wearable sensor |
2022[73] | Cellulose Nanofiber-Reinforced MXene Membranes, PTFE | Mechanical energy | Voc: 1,120 V, Isc: 25 µA, Qsc: 100 µC/m2, Power density: 6.25 W/m2 | Wearable sensor for gesture recognition |
2023[74] | Chemically modified MXene nanoflakes, PVDF-TrFE composite | Mechanical energy | Voc: 110 V, Isc: Not specified, Qsc: Not specified, Power density: ~130 mW/m2 | Energy harvesting, human movement monitoring |
2023[75] | Engineered electrodes with 2D Ti3C2Tx-MXene sheets | Human motion | Voc: 500 V, Isc: Not specified, Power density: Not specified | Biomechanical energy harvesting, self-powered sensing |
2023[76] | MXene embedded in hybrid gel-based polymer (HG-MBP) | Mechanical energy (piezo-triboelectric effect) | Voc: 80 V, Isc: 14 µA, Current density: up to 17.5 mA/m2 | Energy harvesting, gesture recognition |
2019[77] | PVA/MXene nanofibers film | Human movements | Voltage: ~2.9 V (charging a 22 µF capacitor), Power density: 1,087.6 mW/m2 | Body motion monitoring, EWOD chip powering |
2022[78] | PVDF/0.4Ti3CNTx | Mechanical energy | Voc: ~170 V, Current density: 19 mA/m2, Power density: 2.5 W/m2 | Wearable electronics, energy supply |
The role of MXene in triboelectric layer
In TENGs, the triboelectric layer is a critical component that directly influences the device efficiency in generating electrical energy. This layer is responsible for accumulating and transferring electrical charges through contact and separation processes. Integrating MXene into the triboelectric layer has been a significant advancement. High electronegativity and ability of MXene to form versatile composites enhance the triboelectric properties of the friction layer, leading to improved charge generation and energy conversion efficiency.
Improving the electronegativity of the triboelectric layer
Electronegativity in the context of TENGs refers to a tendency of materials to attract and hold onto electrons, a key factor in enhancing triboelectric charge generation. When two different materials come into contact, the one with higher electronegativity gains electrons, becoming negatively charged, while the other loses electrons, becoming positively charged. Introducing MXene, known for its high electronegativity, into the triboelectric layer of TENGs, significantly improves their ability to generate and accumulate triboelectric charges, leading to increased electrical output.
In their transformative work, Jiang et al. developed a highly flexible and efficient TENG utilizing a novel combination of MXene and polydimethylsiloxane (PDMS) composite films, paired with a laser-induced graphene (LIG) electrode[59] [Figure 4A]. This integration of MXene significantly enhanced both the electrical conductivity and the tribo-electronegativity of the TENG, resulting in an output performance seven times greater than that of conventional flat PDMS-based TENGs. Demonstrating considerable adhesion and outstanding flexibility, this TENG has been successfully applied in energy harvesting from leaf swing movements and as an energy-collecting writing board. Furthermore, the research team innovatively developed an M-TENG array serving as a self-powered sensor, adept at handwriting recognition, thus indicating its potential in myriad applications ranging from tactile sensing for pads to sophisticated robotics and human-machine interaction systems.
Figure 4. (A) A graphical representation of the process to create a bendable TENG using a composite film of porous PDMS/MXene alongside an electrode made from laser-induced graphene (LIG)[59]. (Published by Elsevier in 2019). (B) A schematic representation of a versatile TENG apparatus. It includes an SEM image that illustrates the upper and lower electrodes crafted from silver nanowires (AgNWs), with an inset showing AgNWs dispersed in a solvent (scale bar: 1 μm). Additionally, an optical microscopy image of the composite friction film is shown, with an inset displaying the solvent for the composite (scale bar: 200 μm). This is accompanied by a graphical comparison of the absorbance levels between a pure PDMS film and a PDMS/Mxene composite film[60]. (Published by Elsevier in 2021). (C) A diagrammatic depiction of the NDL-TENG[61]. (Published by Elsevier in 2022).
Adding to the advancements in TENG technology, Liu et al. focused on developing a multifunctional and stretchable TENG using PDMS/Mxenes composites as the triboelectric layer [Figure 4B][60]. This TENG was designed to efficiently harvest both mechanical and light energies, signifying a significant leap in the field. Incorporating Mxenes, renowned for their highly electronegative surface and robust surface plasmon excitations, substantially improved the energy conversion efficiency of TENGs, especially under illumination. This was evident as the device showed marked improvements in its open-circuit voltage (Voc) and short-circuit current (Isc) under light exposure, with a notable light power conversion efficiency of 19.6%. The versatility of this TENG was further underlined by its capability in environmental sensing, where it could adeptly detect sound instructions and wind speed, thereby offering broad insights across various settings.
Research by Rahman et al. contributed significantly to the field with the development of a novel double-layered TENG that incorporated a composite of metal-organic framework-derived nanoporous cobalt oxide (NPCO)/silicone and MXene/silicone [Figure 4C][61]. This design showed a notable improvement in energy harvesting efficiency. The integration of MXene amplified the electronegativity of the nanocomposite by nine times, which was instrumental for the enhanced performance of TENGs. The design also included innovative micro/nanostructures to increase hydrophobicity and water resistance, while integrating conductive knitted fabric as a flexible electrode added significant stretchability (230%) and durability to the device. The high sensitivity (5.82 V/kPa) of the device made it exceptionally suitable as a self-powered biomotion sensor, applicable in various devices including foot pressure distribution sensor arrays and self-powered wearable keyboards.
Increasing charge density
Dielectric constant of a material, a measure of its ability to store electrical energy in an electric field, directly influences the charge density that can be induced on the surface of a TENG during operation. High dielectric constant materials can support a larger induced charge under the same mechanical deformation, thereby increasing the output voltage and current of the TENG. The concept of charge trapping further complements this by providing a mechanism for maintaining the separated charges at the interface of the TENG materials, even when the external mechanical force is removed. Charge trapping sites, which can be intrinsic defects or intentionally introduced dopants within the materials, capture and hold onto the charges, preventing their immediate recombination. This capability not only enhances the immediate output of the TENG but also contributes to a more stable and sustained energy generation over time, as the trapped charges can be released slowly or under specific conditions, ensuring a consistent output. MXenes, with their unique layered structure and high surface area, offer an exceptional capacity to enhance charge density. By incorporating MXene into TENGs, researchers have been able to create devices with increased charge retention capabilities and higher transfer charges, leading to more efficient energy harvesting.
In the study by Kim et al., a novel approach to enhancing wearable TENGs is presented by developing all-printed, sustainable wearable TENGs based on electron accumulation polymers (EAPs)[62]. Central to this innovation is using MXene materials, specifically Ti3C2Tx, which are crucial in achieving superior charge retention and volumetric charge density [Figure 5A]. This exceptional performance is attributed to a three-step electron-trapping mechanism involving electron transfer and transport from polytetrafluoroethylene (PTFE)/single-walled carbon nanotube (SWCNT) and electron trapping in MXene, with the electron density trapped in MXene measured at approximately 27 nC cm-2 under a negative bias voltage. The TENGs, fabricated via a cost-effective screen-printing process on a stretchable substrate, exhibit remarkable mechanical stability and durability, crucial for real-world applications.
Figure 5. (A) A schematic outline detailing the sequential layering in a TENG made from electroactive polymers (EAPs) designed for charge accumulation[62]. (Published by Elsevier in 2022). (B) A schematic representation of the TENG architecture that incorporates TPU/mica and PVDF/MXene nanofibers[63]. (Published by Elsevier in 2023). (C) Depiction of the SF@MXene-A-based TENG structure, highlighting that the effective interaction area between SF@MXene-A and the PDMS sponge measures 4 cm2, constituting a square with each side being 2 cm in length[64]. (Published by ACS in 2023). (D) A three-dimensional schematic of the EN-TENG, complemented by an FESEM image showcasing Nylon-11 nanofibers alongside PVDF-TrFE/MXene nanofibers[65]. (Published by ACS in 2021).
Li et al. have innovatively designed a high-performance TENG leveraging the unique properties of MXene materials [Figure 5B][63]. This novel combination, paired with polyvinylidene fluoride/MXene (PVDF/MXene) nanofibers, has resulted in a notable increase in transferred charge, reaching 82.4 nC, compared to 38.6 nC from pure thermoplastic polyurethane (TPU) nanofibers. The TENGs fabricated with TPU/mica nanofibers exhibit a remarkable power density of 1,458 mW/m2, a 16-fold improvement over TENGs based solely on TPU nanofibers. Beyond their impressive performance in energy harvesting, these TENGs also demonstrate great potential in wearable self-powered sensors.
Tan et al. have engineered a novel high-performance, biocompatible porous TENG by integrating silk fibroin (SF) with MXene to create a composite aerogel (SF@MXene-A)[64]. This innovative approach significantly enhances the performance of TENGs through the MXene addition, which notably increases the specific surface area and surface charge density of the aerogel [Figure 5C]. The design of SF@MXene-A achieves a remarkable Voc of 545 V and a maximum Isc of 16.13 μA, which is 2-3 times higher than the best values reported for other aerogel-based TENGs. This advancement in TENG technology is a testament to the efficiency of incorporating MXene into SF, a sustainable biomaterial, thereby enhancing the charge generation capacity due to the interconnected micropore structure and rich surface functional groups of MXene. High electronic conductivity, mechanical strength, and hydrophilicity of MXene, along with its abundant surface functional groups (-O, -F, -OH), significantly contribute to the increased charge density and improved output performance of the TENG.
Rana et al. have designed an advanced electrospun nanofiber-based TENG employing a PVDF-trifluoroethylene (TrFE)/MXene nanocomposite, characterized by its high dielectric constant and significant surface charge density [Figure 5D][65]. The incorporation of MXene nanosheets into the PVDF-TrFE polymer matrix significantly boosted the electronegativity of the composite, leading to a fourfold increase in the output of TENGs that can be attributed to the dual role of MXene: increasing surface charge density through its capacitive effect and reducing it via conductive leakage. The as-designed TENG was successfully utilized as a self-powered switch in smart home systems, controlling appliances such as fire alarms, fans, and smart doors.
The role of MXene in TENG’s electrode layer
The electrode layer in TENGs plays a vital role in collecting and transferring the electrical charges generated in the triboelectrification layer. The traditional electrode layer has several potential shortcomings. One common issue is the durability and stability of these electrode layers. They degrade over time due to environmental factors such as temperature changes, humidity, or chemical reactions, affecting the longevity and reliability of the devices in which they are used[79-82]. The integration of MXene into the electrode layer has been transformative, offering a combination of high electrical conductivity and mechanical durability. These characteristics are crucial for creating efficient electrodes that can withstand mechanical stress while maintaining excellent charge transfer capabilities. Versatility of MXene in forming composites also allows for developing electrodes with additional properties, such as self-repair and stretchability, further broadening the scope of TENG applications in flexible and wearable electronics.
Stable performance of MXene-based electrode
The electrode layer in TENGs is crucial for efficiently collecting and transferring the generated triboelectric charges. Fan et al. innovatively utilized Ti3C2 MXene, integrated with cotton fabric, to develop a novel, highly flexible TENG, named MC-TENG, serving as a self-powered sensor[66]. MXene imparts electrical conductivity and negativity, while the fabric ensures strength and flexibility [Figure 6A]. This MXene-based electrode layer is pivotal in the functionality of TENGs, as it loses electrons upon frictional contact with its silicone rubber friction layer, generating up to 400 V of Voc through hand press-release cycles. A standout feature of the MC-TENG is its ability to accurately sense the mass of steel objects (ranging from 2 to 200 g) with consistent repeatability and a linear relationship between mass and signal.
Figure 6. (A) The design involving the encapsulation of a TENG, incorporating two layers for friction and a singular electrode layer. (Published by Elsevier in 2023). (B) A detailed schematic of the TENG sensor that utilizes an MPP film. (Published by Elsevier in 2021). (C) A schematic representation of the charge distribution within the CM-TENG, attributed to the effect of contact triboelectrification[68]. (Published by AFM in 2020).
The work by Zhang et al. has addressed a critical challenge in developing TENGs in ensuring stable charge transfer between electrode and triboelectric layers [Figure 6B][67]. Utilizing MXene materials, they innovatively designed an integrated MXene-PEDOT: PSS/PTFE (MPP) film, serving both as the electrode and triboelectric layer, fabricated through a straightforward vacuum-assistant filtration method. This TENG-based sensor, distinguished by its remarkable sensitivity to minute forces (exceeding 6.05 V/N), demonstrates rapid response and recovery times (52 and 34 ms, respectively) and maintains exceptional stability over 6,000 cycles. The study showcases the efficacy of MXene nanosheets in creating a flexible TENG with an integrated film, where the seamless connection between the electrode and triboelectric layer enhances charge transfer efficiency, resulting in high output performance.
In their innovative research, Cao et al. developed the CM-TENG, a stretchable shape-adaptive TENG, using an MXene liquid electrode [Figure 6C][68]. This TENG integrates cellulose nanofibers (CNFs) and MXene nanosheets, leveraging exceptional electron-trapping properties of MXene. The device shows remarkable electrical output with Voc of approximately 300 V and Isc of around 5.5 μA, even under extreme deformations such as stretching, twisting, and folding. The combination of soft materials such as silicone rubber with the MXene-based liquid electrode ensures the durability and flexibility of CM-TENG. Its ability to light up 230 LEDs and charge capacitors for wearable electronics demonstrates its potential in applications including soft robotics, human-machine interaction, green energy, and self-powered sensors.
Self-repair and high stretchability of MXene-based electrodes
The advent of wearable and flexible electronics has necessitated the development of self-healing and stretchable components. MXene electrodes in TENGs have emerged as a leading solution in this realm. These electrodes are not only highly conductive but also possess intrinsic self-repairing capabilities and exceptional stretchability. This makes them ideal for TENG applications that require durability and flexibility, such as wearable devices and electronic skins. The integration of MXene into TENG electrodes has thus paved the way for more resilient and adaptable energy harvesting technologies.
In our study, the advancement in wearable electronics is exemplified by the development of a self-powered TENG designed by Cai et al., which demonstrates unprecedented self-healing capabilities and remarkable stretchability exceeding 760% [Figure 7A][69]. This TENG incorporates a meticulously designed double-crosslinked PDMS (DCS-PDMS) matrix, achieving a synergistic balance between imine and hydrogen bonds (H-bonds), which is pivotal for its self-healing efficacy and mechanical robustness. The DCS-PDMS matrix enables the TENG to recover from damage within 2 h, maintaining its original triboelectric performance after repeated cycles of mechanical deformation. The inclusion of MXene as a core component of the conductive composite in the electrode layer significantly contributes to the functionalities of TENGs. This composite leverages H-bonds formed between MXene flakes and lightly cross-linked PDMS to ensure durable conductivity and effective self-repair capabilities. Importantly, this research demonstrates the role of MXene-based composites in sustaining consistent electrical performance under extreme mechanical conditions, including stretchability beyond 760%, without compromising its self-healing efficiency. Such remarkable attributes underscore the potential of MXene materials in revolutionizing wearable electronics by offering robust, self-healing, and highly stretchable energy-harvesting devices.
Figure 7. (A) A schematic outlining the chemical structure[69]. (Published by Elsevier in 2020). (B) The synthesis process for an MXene-based composite hydrogel, alongside the architectural design of both FS-TENG and SC[70]. (Published by Elsevier in 2023). (C) A detailed schematic showing the MXene/PVA hydrogel as synthesized, highlighting its primary and secondary cross-linking networks[71]. (Published by AFM in 2021). (D) A graphical illustration detailing the production method for a stretchable and transparent MAMP-TENG[72]. (Published by Elsevier in 2020).
Groundbreaking work by Wang et al. on a self-charging power package introduces a novel MXene-based polyacrylamide (PAM) hydrogel, which is pivotal in revolutionizing wearable and implantable electronic devices due to its dual functionality [Figure 7B][70]. This MXene-infused hydrogel not only acts as an electrolyte in all-solid-state SCs but also serves as the electrode in a self-powered TENG, seamlessly integrating energy harvesting and storage capabilities. The hydrogel distinguishes itself by exhibiting unparalleled mechanical stretchability and excellent ionic conductivity, traits that are essential for the flexibility and durability of wearable electronics. Furthermore, its biocompatibility and high electrochemical performance, as demonstrated through implantation experiments in rats, underscore the potential of MXene materials in advancing bioelectronics. The ability of the hydrogel to maintain high capacitance and long-term stability, even when stretched or implanted, signifies a significant step forward in developing self-sufficient, bio-integrated electronic systems.
In their groundbreaking work, Luo et al. introduced the MXene/polyvinyl alcohol (PVA) hydrogel-based TENG (MH-TENG), a pivotal advancement in flexible, portable, and self-powered electronic devices[71]. This innovation leverages the unique properties of MXene nanosheets to significantly enhance the structural integrity and functionality of the PVA hydrogel matrix. The integration of MXene nanosheets promotes robust crosslinking within the hydrogel, substantially augmenting its stretchability [Figure 7C][71]. Remarkably, the MH-TENG demonstrates an ability to be stretched up to 200% of its original length, maintaining a consistent increase in short-circuit voltage proportional to its stretchability. This feature is critical for applications requiring durability and flexibility, such as wearable movement monitors and energy harvesting devices. The self-healing capabilities of the MXene/PVA hydrogel are equally notable, demonstrating rapid and seamless recovery upon damage. This self-repairing feature ensures the longevity and reliability of the MH-TENG under various conditions, making it an ideal candidate for sustainable and durable electronic applications. The combined attributes of exceptional stretchability, enhanced conductivity, and self-repairing capabilities highlight the potential of MH-TENG to revolutionize the field of wearable electronics and energy harvesting, paving the way for innovative applications that require materials with high endurance and adaptability.
Liu et al. have ingeniously devised a stretchable and transparent TENG by employing a MXene-silver nanowires (AgNWs)-MXene-polyurethane (PU) nanofibers (MAMP) electrode, showcasing a strategic use of industrial-grade electrospinning and vacuum filtration techniques [Figure 7D][72]. The architecture of MAMP-TENG, featuring a layered structure of vacuum-filtered Ti3C2Tx (MXene) nanosheets and AgNWs interlaced on PU nanofibers, not only fortifies the AgNWs network through enhanced mechanical interlocking but also significantly bolsters the adhesion of the network to the PU nanofibers via hydrogen bonding facilitated by MXene. This intricate assembly process yields an electrode with remarkable stretchability, capable of enduring up to 180% strain, and demonstrates excellent durability, maintaining its electrical properties after 1,000 stretch-release cycles at 65% strain. Furthermore, the MAMP electrode preserves its functional integrity and optoelectronic properties after deformation, indicating its high resilience and suitability for applications in self-powered wearable sensors and energy harvesters. The synergistic effect of the nanofiber scaffold reinforcement and the adhesive properties of MXene not only contributes to the high stretchability and durability of the electrode but also ensures the retention of its functionality after stretching, making it a promising component for the next-generation wearable electronics and human-machine interfaces.
Engineering the triboelectric interface
Enhancing the frictional electrical output of TENGs is crucial for optimizing their energy harvesting efficiency. This enhancement involves not just selecting high-performance materials but also innovative design approaches and engineering techniques. MXene, with its exceptional properties, plays a central role in this advancement. Its integration into TENGs through various methods improves the electrical output, making these devices more efficient and versatile for a range of applications.
Physical and chemical modification
The physical and chemical modification of materials in TENGs directly influences their ability to generate and maintain electrical output. By altering the surface properties and internal composition of materials, researchers can significantly improve the performance of TENGs. Incorporating MXene into TENGs through various physical and chemical modification techniques enhances their electrical output by improving surface roughness, charge density, and electron affinity. These modifications enable TENGs to generate higher power from the same mechanical input, broadening their application in energy harvesting and self-powered devices.
The field of TENGs has seen remarkable advancements due to the incorporation of MXene materials, with innovative research by Xing et al. laying a significant foundation[73]. They developed a unique class of MXene films enhanced by CNFs, forming a spider-web-like structure through a vacuum-assisted filtration process [Figure 8A]. These MXene/CNF composites, exhibiting remarkable flexibility and stability, stand out in TENG applications. When combined with PTFE, the MXene/CNF films showed impressive electroactivity, particularly excelling in TENG applications with an output voltage exceeding 1,120 V and a surface charge density of 100 μC m-2. This high performance is attributed to the microstructures on their rough surfaces and the presence of polar terminations on the MXene, crucial for enhancing triboelectric effects and long-term stability.
Figure 8. (A) Depicts the architecture of current MXene-based TENGs[73]. (Published by ACS in 2022). (B) Illustrates the chemical alteration of Ti3C2 through hydrothermal methods and the creation of layers for positive and negative friction[74]. (Published by Elsevier in 2023). (C) Provides a schematic of the synthesis technique for Transition Metal Sulfides (TMSs)[75]. (Published by Elsevier in 2023). (D) Offers a comprehensive illustration of the HG-MBP fabrication, which includes an MBP composite film applied via spin coating onto an aluminum electrode, itself attached to a polyimide (PI) substrate. An inset demonstrates the flexibility of the HG-BMP that has been produced[76]. (Published by Elsevier in 2023).
Building on this foundation, Cao et al. introduced a novel method for enhancing TENGs through the innovative use of chemically modified MXene materials, specifically Ti3C2 nanoflakes [Figure 8B][74]. Integrating these nanoflakes into polymer matrices, they created composite friction layers with either positive or negative triboelectric properties. By incorporating N-functionalized Ti3C2 (N-Ti3C2) with PVDF-TrFE and NH2-functionalized Ti3C2 (NH2-Ti3C2) with Nylon 11, they developed friction layers that not only increased the surface potential but also enhanced it further through electrical polarization. This strategic composition resulted in TENGs with impressive electrical outputs and mechanical strength, maintaining consistent output over 14,400 cycles. This breakthrough underlines the crucial role of MXene materials in advancing TENG technology, offering a facile and effective method for fabricating high-performance TENGs with enhanced electrical and mechanical properties.
Expand collection channels
Expanding collection channels in TENGs refers to increasing the pathways and mechanisms through which electrical charges are generated and transferred. This can be achieved by designing multi-layer structures or combining the output of piezoelectric and frictional electricity. Versatile nature of MXene allows it to be used in various configurations within TENGs, thereby expanding the collection channels.
In the study conducted by Anwer et al., a novel TENG is developed, leveraging the unique properties of MXene materials, specifically micron-sized Ti3C2Tx-MXene ultrathin sheets (TMSs), to enhance its power density and durability[75]. This TENG employs a layer-by-layer stacked vertical CS mode design, integrating TMSs into a polyethylene terephthalate (PET)-based tribo-negative electrode and a NaCl-enhanced PVA matrix as a tribo-positive layer [Figure 8C]. The strategic incorporation of TMSs, known for their high dielectric constant and charge trapping capabilities, results in a synergistic effect, achieving Voc of approximately 390 V, Isc of around 96 μA, and a power density of 6.66 W m-2. The role of TMSs in charge accumulation and trapping increases charge separation and overall output power. Furthermore, their effectiveness in monitoring sensitive physiological human movements confirms their utility in biomechanical energy harvesting.
Yun et al. have innovatively designed a triboelectric and piezoelectric hybrid generator (HG-MBP), incorporating MXene and barium titanate ceramic filler into a PDMS matrix [Figure 8D][76]. MXene serves as a bifunctional conductive filler, enhancing the electrical performance of generators significantly. A 3D-printed robotic hand, mirroring human finger movements, was effectively controlled using the HG-MBP, showcasing its potential as an advanced human gesture manipulation system. Moreover, the implementation of a material detection system utilizing the k-mean clustering method further highlights the versatility of HG-MBP, achieving an impressive classification accuracy of 93.33% in differentiating materials.
Increase stability
MXenes, with their exceptional electrical conductivity and mechanical robustness, offer a dual advantage: they improve the electrical performance and durability of TENGs under repeated use. Firstly, their high electrical conductivity facilitates efficient charge transfer within TENG devices. This ensures that the electrical energy generated from mechanical movements is quickly and effectively captured, reducing energy loss and improving the overall efficiency of the device. Additionally, their rapid charge transfer capability minimizes the charge accumulation at the interfaces, which can lead to electrical fatigue and degradation of materials over time. By enabling a more efficient charge flow, MXenes help maintain the performance of TENGs across a broader range of operational conditions and over extended periods. Secondly, the mechanical strength and flexibility of MXene materials contribute to the physical stability of TENG devices. MXenes can withstand repeated mechanical deformations without significant wear or structural failure, a critical attribute for TENGs that rely on continuous physical interactions to generate electricity. This mechanical resilience helps preserve the integrity and alignment of TENG components, ensuring consistent output even under high strain or after many cycles of operation.
The research by Jiang et al. introduces an elf-powered device utilizing the unique properties of MXene materials[77]. They integrated highly electronegative and conductive MXene nanosheets with PVA to create electrospun nanofiber films, forming a flexible TENG with high stability [Figure 9A and B]. The MXene nanosheets enhanced the electrical performance of the device, and the ability of TENGs to efficiently harvest electrical power and its application in powering electrowetting on dielectric (EWOD) chips for droplet transport indicate its potential in areas such as drug delivery, DNA analysis, and environmental monitoring.
Figure 9. (A) A detailed schematic depicting the creation of a TENG using a film composed of PVA/MXene and silk nanofibers. (B) Assessment of the PVA/MXene nanofiber film's stability in a TENG configuration, subjected to a consistent 10 N compressive force at a frequency of 10 Hz, demonstrating durability over 124,000 cycles. Inset shows a zoomed-in section of the performance curve[77]. (Published by Elsevier in 2019). (C) The manufacturing steps for a TENG with an all-fiber structure. (D) Voltage generation by the all-fiber TENG, analyzed through a continuous contact-separation method, across approximately 14,000 cycles[83]. (Published by ACS in 2021). (E) A schematic representation of a TENG device fabricated using either PVDF/Ti3CNTx or PVDF/Ti3C2Tx films[78]. (Published by Elsevier in 2022).
Building on work by Huang et al. made a significant contribution to wearable electronics with the design of an all-fiber-structured TENG, addressing the limitations of conventional power sources[83]. This TENG, fabricated through a one-pot electrospinning technique, featured a unique combination of ethyl cellulose/polyamide 6 (EC/PA6) and MXene-enhanced PVDF nanofibers as triboelectric positive and negative materials, respectively [Figure 9C and D]. The MXene materials, notable for their strong electronegativity and conductivity, played a pivotal role in boosting the output and stability of TENGs, reaching peak power densities of 290 mW/m2 at a 100 MΩ load resistance and long-term stable output.
Complementing these innovations, Song et al. meticulously designed a TENG using an innovative dielectric modulation approach that significantly enhanced its output performance, pivotal for practical applications in portable and distributed power sources [Figure 9E][78]. The advancement centered around strategically integrating Ti3C2Tx and Ti3CNTx MXene materials into PVDF ferroelectric polymers. This integration fostered a marked increase in the charge trapping capacity and surface charge density, showcasing unique properties of MXenes, especially in Ti3CNTx, where nitrogen atoms partially substitute carbon atoms. This substitution introduced an array of surface-terminating groups, enhancing the interfacial interactions and compatibility with the PVDF matrix and locking in dielectric polarization. As a result, the PVDF/Ti3C2Tx and PVDF/Ti3CNTx films exhibited a significant boost in dielectric modulation, leading to a remarkable improvement in TENG device performance.
COMPREHENSIVE APPLICATION OF M-TENG
Applications of M-TENG in extreme environments
Extreme environments pose unique challenges for electronic devices, demanding high durability, resilience, and adaptability. TENGs based on MXene, with their inherent robustness and flexibility, are well-suited for such conditions. Their ability to harvest energy from environmental mechanical motions and convert it into electrical energy, even under harsh conditions, makes them ideal for applications in aerospace, deep-sea exploration, and outdoor energy harvesting [Table 2].
The performance of M-TENG
Data | Material composition | Energy source | Output | Applications |
2021[84] | Carbon nanotube (CNT) - MXene composite | Structural health monitoring | Voc: ~50 V, Isc: ~1.2 µA, Power density: Not specified | Structural health monitoring systems (Extreme environment application) |
2023[85] | MXene-PVDF nanocomposite | Mechanical energy | Voc: ~150 V, Isc: ~10 µA, Power density: ~22.5 mW/m2 | Wearable electronics, energy harvesting (Extreme environment application) |
2021[86] | PMC nanofibers, Nylon 6/6 | Mechanical motion | Voc: 724 V, Isc: 163.6 µA, Qsc: 182 nC | Extreme environment application |
2022[87] | Ethylene chlorotrifluoroethylene, Hydrogel | Human movements | Voltage: ~170 V, Current density: 19 mA/m2, Power density: 2.5 W/m2 | Marine Applications |
2023[88] | PVA/MXene, PVDF | Ocean, Humidity | Voc: 1,056 V, Isc: 36.6 µA | Water sterilization (98.99% efficiency), Energy harvesting |
2023[89] | MXene/SiO2@PDMS | Droplet energy | Voc: 152 V, Isc: 5.8 µA, Peak power: 1.01 × 10-4 W | Energy harvesting, Cathodic protection systems (Marine applications) |
2022[90] | PPUH-m-MXene | Mechanical motion | Voc: ~310 V, Isc: 29 µA, Power density: ~1,150 mW/m2 | EMI shielding, Self-powered sensing, Wearable sensors |
2022[91] | Ecoflex@MOF-525, Ecoflex@MXene@Co-NPC | Biomotion | Sensitivity: 149 V/kPa, Power density: 25.7 W/m2 | Wearable biomotion monitoring, Handwriting recognition, Self-powered tactile sensors |
2023[92] | Composite hydrogel with polyacrylamide, hydroxypropyl methylcellulose, MXene | Biomechanical | Voltage: 183 V, Power density: 78.3 mW/m2 | Miniature electronics, Strain sensors |
2023[93] | Ionic hydrogel (PTSM) with PAM, TA, SA, MXene | Mechanical | Power density: 54.24 mW/m2 | HMI systems, Gesture recognition, Robot control |
2023[94] | Carbon nanotube (CNT) - MXene composite | Mechanical energy (structural monitoring) | Voc: ~50 V, Isc: ~1.2 µA | Structural health monitoring systems |
2023[95] | MXene-PVDF nanocomposite | Mechanical energy | Voc: ~150 V, Isc: ~10 µA, Power density: ~22.5 mW/m2 | NH3 detection |
2021[96] | PMC nanofibers, Nylon 6/6 | Mechanical motion | Voc: 724 V, Isc: 163.6 µA, Qsc: 182 nC | NH3 detection |
2022[97] | Ethylene chlorotrifluoroethylene, Hydrogel | Human movements | Voltage: ~170 V, Current: 19 mA/m2, Power density: 2.5 W/m2 | Formaldehyde detection |
2022[98] | PVA/MXene, PVDF | Ocean, Humidity | Voc: 1,056 V, Isc: 36.6 µA | Ethanol detection |
2022[99] | MXene/SiO2@PDMS | Droplet energy | Isc: 5.8 µA, Voc: 152 V, Peak Power: 1.01 × 10-4 W | Tactile sensing |
2023[100] | PPUH-m-MXene | Mechanical motion | Voc: ~310 V, Isc: 29 µA, Power: ~1,150 mW/m2 | Tactile sensing |
2023[101] | Ecoflex@MOF-525, Ecoflex@MXene@Co-NPC | Biomotion | Sensitivity: 149 V/kPa, Power density: 25.7 W/m2 | Hydrogen production |
2023[102] | Composite hydrogel (polyacrylamide, hydroxypropyl methylcellulose, MXene) | Biomechanical | 183 V, Power density: 78.3 mW/m2 | Cathodic protection |
2023[103] | Ionic hydrogel (PTSM) with PAM, TA, SA, MXene | Mechanical | Power density: 54.24 mW/m2 | Cathodic protection |
High impact resistance and durability of M-TENG
The advancements in high-impact resistance and durability of TENGs based on MXene are notable for their potential to revolutionize clean energy harvesting and storage, especially in demanding environments.
Deka et al. developed a woven carbon fiber (WCF)-based TENG cum structural SC, epitomizing this innovation [Figure 10A][84]. This multifunctional device seamlessly blends high load-bearing capacity with resilience under extreme conditions, a critical requirement for outdoor applications. Its energy conversion efficiency, an impressive 84%, with a power output of 8.9 W/m2, demonstrates the efficacy of integrating MXene into the structure. The robust mechanical strength and impact resistance of this device make it a promising candidate for applications in self-charging automobiles, unmanned aerial vehicles, and sectors such as aerospace, sensors, and electronics.
Figure 10. (A) Depicts the synthesis method for ZnCuSe2 nanoporous material on a Woven Carbon Fabric (WCF) and the subsequent development of a multifunctional device using Vacuum Assisted Resin Transfer Molding (VARTM) and a spin-coating technique[84]. (Published by Elsevier in 2021). (B) Outlines the fabrication process for Polyvinylidene Fluoride (PVDF)-MXene nanocomposite fibers[85]. (Published by Small in 2023). (C) Shows the chemical structure and illustrates the impact of incorporating MXene into the PVDF matrix, leading to hydrogen bonding due to a synergistic effect[86]. (Published by Elsevier in 2021).
In parallel, Hasan et al. have pioneered the scalable fabrication of Ti3C2Tx MXene-embedded PVDF nanocomposite fibers through a thermal drawing process, representing a significant stride in textile-based energy harvesting and sensing technologies [Figure 10B][85]. These fibers, when woven into fabric TENGs, not only exhibit enhanced electrical output but also maintain flexibility, essential for large-area applications. The fabric TENGs, with a power density of 40.8 mW/m2, demonstrate durability over 12,000 cycles. This durability is crucial for long-term applications, particularly in extreme environments where reliability is paramount.
Further enhancing the scope of M-TENGs, Bhatta et al. introduced a PVDF/MXene composite nanofiber, marking a significant advancement in triboelectric energy harvesting. By incorporating MXene materials, specifically Ti3C2Tx nanosheets, into the PVDF matrix, they achieved a 270% increase in dielectric constant and an 80% surge in surface charge density [Figure 10C][86]. This fusion substantially improved the triboelectric capabilities of nanofibers, yielding a peak power output of 4.6 mW and a power density of 11.213 W/m2 at a 2 MΩ load. The durability of this TENG, demonstrated over 60,000 cycles, along with its ability to effectively charge storage capacitors and power low-energy electronics and commercial LEDs, highlights the potential of MXene-enhanced TENGs in various real-world applications.
Marine applications of M-TENG
Marine applications of TENGs based on MXene materials have shown significant advancements, particularly in the realm of environmental monitoring and energy harvesting in marine environments. In their innovative work, Wang et al. developed a wave-driven liquid-solid TENG, utilizing an ethylene chlorotrifluoroethylene (ECTFE) film coupled with ionic hydrogel electrodes[87]. This design excels in harvesting wave energy, a critical component in marine applications [Figure 11A]. A pivotal aspect of this development is integrating a novel self-powered MXene/TiO2/SnSe sensor specifically tailored for detecting sulfur dioxide (SO2) gas. The sensor exhibited a remarkable response, showcasing an enhancement over traditional resistive sensors by a significant factor.
Figure 11. (A) Both the structural schematic and an actual photograph of a liquid-solid TENG[87]. (Published by Elsevier in 2022). (B) A detailed schematic diagram showcasing the preparation method and the cross-linking mechanism involved in creating a PVA/MXene composite film[88]. (Published by Elsevier in 2023). (C) A flow chart outlining the production process for MXene/SiO2@PDMS composite material[89]. (Published by Elsevier in 2023).
From these developments, Sun et al. focused on enhancing the efficiency of blue energy harvesting in harsh sea-level environments by optimizing the MXene doping ratio in a PVA triboelectrode [Figure 11B][88]. The strategic inclusion of MXene introduces additional hydrogen bonding sites on the PVA surface, attracting more water molecules and thereby increasing the electropositivity of the material. This enhancement significantly boosts the anti-fouling capabilities of the material by forming a hydration layer, an essential feature in marine applications where biofouling can significantly hamper device performance. Moreover, incorporation of MXene also contributes to forming microcapacitors, enhancing the dielectric properties of the material. The charge density and binding energy of PVA/MXene and PVA, both before and after water molecule adsorption, not only showcase superior triboelectric properties and remarkable stability under marine environmental conditions but also offer a promising avenue for applications in water research, energy harvesting, sewage treatment, and beyond.
Traditional hydropower generation methods are less effective in low-frequency energy scenarios, where TENGs come into play. In another innovative study, Wang et al. tackled the challenge of efficiently harvesting raindrop energy, a key area in sustainable energy research, especially pertinent in marine contexts where water abundance is a given[89]. By integrating high dielectric constant MXene and hydrophobic nano-SiO2 into PDMS, the researchers could significantly amplify the permittivity and triboelectric performance of the composite [Figure 11C]. The strategic use of SiO2 not only improves the dielectric properties and charge density of the composites but also enhances the hydrophobicity and surface roughness of PDMS, facilitating quicker droplet movement and stabilizing TENG performance in high humidity conditions. The MXene/SiO2@PDMS composites, synthesized through a physically doped method, display increased electronegativity and conductivity, elevating their effectiveness in raindrop energy harvesting, highlighting the potential of composites for green energy harvesting and self-powered sensing and offering new avenues for practical TENG applications in electrochemical cathodic protection.
Self-Repair and high tensile capacity of M-TENG
The development of self-repairing and high tensile capacity TENGs based on MXene represents a groundbreaking advancement in the field of flexible electronics and sustainable energy harvesting.
Du et al. have made a significant contribution with their design of an ultra-flexible and self-healing TENG using modified Ti3C2Tx MXene-based nanocomposite elastomers [Figure 12A][90]. The strategic incorporation of modified MXene enhances the electronegativity of the device, which is pivotal in achieving these high-performance metrics. Additionally, this TENG exhibits superior electromagnetic interference (EMI) shielding capabilities, achieving a maximum shielding effectiveness of 48.1 dB in the X-band. The enhanced shielding is predominantly attributed to electromagnetic absorption due to significant conduction loss within the m-MXene network, multiple reflections between m-MXene sheets, and a polarization effect on their surfaces. The intrinsic healing ability of sensors, with a healing efficiency of 98.2%, underscores the practicality and durability of the device.
Figure 12. (A) A schematic representation of the PPUH-mMXene TENG fabrication process. This process involves the use of two PPUH-m-MXene nanocomposite elastomers as the triboelectric layers, separated by a pure MXene electrode with a thickness of approximately 50 micrometers[90]. (Published by Elsevier in 2022). (B) A detailed schematic of the M-TENG, showcasing its design and operational principle[91]. (Published by Elsevier in 2022). (C) A diagram illustrating the preparation steps for a PAM/HPMC/MXene hydrogel, highlighting the materials and method involved[92]. (Published by ACS in 2023). (D) The structural schematic of the PTSM-TENG, providing insight into its configuration and components[93]. (Published by ACS in 2023) (E) A schematic presentation of the MXene and PMXene fabrication processes, including a diagram of the wet-spinning procedure used in their creation[94]. (Published by Elsevier in 2023).
Rana et al. developed a high-performance multilayered TENG, characterized by its stretchability and humidity resistance, intended for innovative self-powered biomotion and tactile sensing applications
Furthermore, Li et al. have developed a composite hydrogel comprising PAM, hydroxypropyl methylcellulose, and notably MXene (Ti3C2Tx) nanosheets, revolutionizing the realm of wearable electronic devices [Figure 12C][92]. This hydrogel forms a stable double-chain structure through hydrogen bonding, endowing it with extraordinary qualities such as high strength, enhanced stretchability, superior electrical conductivity, and heightened strain sensitivity. Utilizing this hydrogel, they created a flexible multifunctional TENG (PHM-TENG) that serves as a functional electrode capable of harvesting biomechanical energy. The inclusion of MXene materials is pivotal as their metallic conductivity and hydrophilicity substantially boost the electrical conductivity and strain sensitivity of the hydrogel, while their rich surface functional groups strengthen its mechanical properties. This hydrogel is thus transformed into an effective strain/stress sensor, capable of monitoring mechanical strains such as human motion, with high tensile and stress-strain characteristics.
Innovative work by Zhang et al. on the multifunctional PTSM hydrogel, combining polypropylene amine, tannic acid, sodium alginate, and notably MXene, further underscores the versatility of MXene in flexible electronics[93]. This hydrogel exhibits exceptional stretchability (over 4,600% strain), adhesion, and self-healing capabilities, attributes significantly enhanced by high strain sensitivity and electrical conductivity of MXene [Figure 12D]. Rich surface functional groups of MXene contribute to a negatively charged hydrophilic surface, amplifying the number of reversible H-bonds and thus improving the mechanical properties and self-healing efficiency of the hydrogel. This system, bolstered by integrating machine learning techniques, achieves an impressive 98.7% accuracy rate in object classification and recognition.
Finally, research by Hao et al. in designing an innovative energy device that caters to the demanding requirements of wearable products and soft electronics highlights the transformative impact of MXene materials[94]. The core of this breakthrough lies in the novel use of MXene materials, specifically the dopamine-modified MXene (P-MXene) combined with MXene/TPU (MP) fibers to create a homogeneous wet-spun fiber termed MMP [Figure 12E]. The MMP fiber exhibits remarkable properties: an extraordinary stretchability of approximately 675%, robust conductivity (4.32 S/cm), and reliable stability under significant deformation. These characteristics stem from the synergistic combination of superior deformation recovery of TPU and consistent electrical conductivity of P-MXene ink. This TENG showcases impressive electrical output even after 100 bending cycles, with no significant attenuation in voltage signals. This capability underscores the potential of the MMP fiber in energy harvesting and human motion sensing applications, providing real-time electrical signal responses.
Application of M-TENG in respiratory detection
The field of medical diagnostics and environmental monitoring has increasingly turned to advanced sensor technologies for non-invasive, real-time detection of various biomarkers and gases. M-TENGs have shown great promise in this realm, particularly for respiratory monitoring and gas detection. Their sensitivity to mechanical movements and ability to generate electrical signals in response to biochemical changes make them excellent candidates for monitoring respiratory patterns and detecting gases such as NH3 and formaldehyde.
Respiratory monitoring and NH3 detection
The advancements in respiratory monitoring and NH3 detection using M-TENGs represent a significant leap in medical technology and environmental sensing. In their pioneering study, Chen et al. explored using MXene materials as functional fillers in developing PVDF-TrFE/MXene composite nanofibers films (PMNFs) [Figure 13A][95]. This innovation demonstrates a considerable enhancement in the triboelectric properties of PVDF-based negative dielectric layers by including MXene fillers, which are highly electronegative. The enhancement in performance is attributed to the improved electron-withdrawing capability and dielectric properties of the films, and the practical application of this research is exemplified in designing a tremor-based TENG integrated into a respiratory monitor, showcasing the potential of PMNFs in real-time respiratory monitoring.
Figure 13. (A) Displays a 3D schematic of the PMM-TENG, providing a comprehensive view of its structure and components[95]. (Published by Elsevier in 2023). (B) Offers a schematic illustration of a self-powered NH3 sensor that operates based on the principles of a TENG[96]. (Published by ACS in 2021). (C) Shows an application diagram for a TENG device based on MXene/NH2-modified Multi-Walled Carbon Nanotubes (MWCNTs), demonstrating its functionality and potential uses[97]. (Published by Elsevier in 2022). (D) Presents the structural design of the HNG, outlining its configuration and the principles behind its operation[98]. (Published by Elsevier in 2022).
A study of Wang et al. further extends the application of MXene in respiratory monitoring, with a focus on NH3 detection[96]. They developed a self-powered sensor utilizing organ-like Ti3C2Tx MXene/metal-organic framework-derived copper oxide (CuO) to detect NH3 at room temperature [Figure 13B]. This sensor is uniquely powered by a TENG based on latex and PTFE, boasting a remarkable Voc of up to 810 V and Isc of 34 μA. The improved NH3 sensing performance of the self-powered sensor based on the MXene/CuO composite is a testament to the enhanced sensing properties systematically discussed by exploring the gas adsorption and desorption process.
Formaldehyde and ethanol detection
The advancements in formaldehyde and ethanol detection using M-TENGs highlight the increasing sophistication and utility of these devices in environmental and health monitoring. In the realm of formaldehyde detection, Wang et al. have made a significant contribution to developing a self-powered sensing system using a TENG[97]. This device is primarily geared towards detecting exhaled gas, underscoring the significant role of MXene materials in enhancing sensor functionality [Figure 13C] The dual-purpose TENG, driven by respiration, serves both as a power source and a sensor within the self-powered system. The MXene/NH2-Multi-Walled Carbon Nanotubes (MWCNTs) composite, acting simultaneously as both the triboelectric layer and the electrode, besides impressive performance metrics, including a peak-to-peak value of Voc of 136 V and output power of 27 μW, its efficacy as a formaldehyde sensor is further highlighted by its excellent gas-sensing response (35% at 5 ppm), a low detection limit of
In the context of ethanol detection, Wang et al. have developed an innovative triboelectric-electromagnetic hybrid nanogenerator (HNG) designed to harness mechanical energy from tire movement and convert it into electrical energy, thereby driving a self-powered gas and motion monitoring system [Figure 13D][98]. The use of Ti3C2Tx MXene/Ag composites plays a crucial role in enhancing the system efficiency and sensitivity, particularly in ethanol detection. The HNG demonstrates an impressive output, with voltages reaching 581 and 62 V at a vehicle speed of 15 km/h for the TENG and electromagnetic generator (EMG), respectively. Significantly, the MXene/Ag-based sensors, fabricated through advanced microelectronic printing and electrospinning techniques, exhibit remarkable sensitivity to ethanol, as indicated by a 204% response at 100 ppm. The application of these sensors extends beyond gas detection to monitoring articular activity of cyclists, offering valuable data for optimizing training and racing strategies.
Multi-applications of M-TENG
The versatility of MXene extends beyond energy harvesting and sensing, finding applications in a wide array of multifunctional devices. This includes tactile sensors, hydrogen production systems, electrochemical cathodic protection, and wearable heaters. The unique electrical, mechanical, and thermal properties of MXene contribute to developing innovative devices that are efficient, flexible, and can be integrated into various platforms, including wearables and portable electronics. This diversification showcases the potential of M-TENGs in contributing to sustainable energy solutions and advanced material applications.
Tactile sensing
The field of tactile sensing has been significantly advanced by incorporating MXene materials into TENGs, leading to groundbreaking developments in touch sensitivity and application diversity. In the pioneering work of He et al., a novel TENG leveraging the unique properties of MXene materials has been developed to enhance energy harvesting and sensing applications[99]. The flexible, self-healing sandwich structural film is composed of two nitrile butadiene rubber (NBR) layers with an intermediate MXene layer [Figure 14A]. This NBR/MXene/NBR film exhibits remarkable self-healing properties, rapidly recovering under a standard hairdryer in 5 s or at skin temperature (~37 °C) over 4 h. The resultant TENG functions as an efficient triboelectric sensor (TS) adept at tracing finger movements and monitoring basketball players' dribbling postures. This capability of converting physical pressure into electrical signals makes it highly suitable for real-time motion tracking in sports and other physical activities.
Figure 14. (A) Describes a TENG crafted from an NBR/MXene/NBR film paired with either PTFE or a basketball epidermis made of PU, highlighting the materials' roles in its operation[99]. (Published by Elsevier in 2022). (B) Offers a schematic representation detailing both the creation and utility of the SPTSA, illustrating the methodological approach and envisioned applications[100]. (Published by Elsevier in 2023). (C) Introduces a Contact-Separation Mode Triboelectric Nanogenerator (CS-TENG), focusing on its principle of operation and design attributes[101]. (Published by AEM in 2023). (D) Provides a schematic overview of a vertically separated FME@TiO2-TENG, elucidating its structural design and functional mechanisms[102]. (Published by Elsevier in 2023). (E) Explains the preparation process for flexible, multifunctional conductive fabrics, detailing the steps involved in their development[103]. (Published by Elsevier in 2023).
Expanding on the versatility of MXene in tactile sensing, Zhi et al. have innovatively designed a biocompatible and antibacterial all-textile structured TENG[100]. This TENG is ingeniously constructed using MXene-doped PVDF (P/M) nanofibers as the tribe-negative component, while antibacterial Ag nanoparticles modified nylon 66 (Ag@nylon 6/6) nanofibers serve as the tribe-positive counterpart
Hydrogen production and cathodic protection
In the realm of renewable energy generation and advanced material applications, using MXene materials in TENGs has led to significant strides, particularly in hydrogen production, electrochemical cathodic protection, and wearable heater development [Figure 14C][101]. Their study pioneers the use of a CS mode TENG, which employs an MXene-incorporated PDMS film as the negative counterpart and demonstrates unprecedented efficiency in converting mechanical energy, derived from simple actions such as hand clapping and toe-tapping, into electrical energy for powering hydrogen and oxygen evolution through water electrolysis.
Advancing further into the field of electrochemical cathodic protection, Nan et al. designed an innovative TENG, the FME@TiO2-TENG, utilizing a composite dielectric structure of melamine foam (MF), MXene, and Ecoflex@TiO2 (FME@TiO2) to significantly enhance electrical output performance [Figure 14D][102]. These micro-capacitances, coupled with inherent strong electronegativity and electrical conductivity of MXene, improve surface charge generation, transfer, and retention within the FME@TiO2 dielectric. The FME@TiO2-TENG demonstrated its utility by serving as a standalone power source for a cathodic protection system in electrochemical applications.
In the work of Yang et al., conductive textile composites including 2D vanadium carbides MXenforms were built with outstanding conductivity and Joule heating properties [Figure 14E][103]. These properties are integral to their application in wearable heaters and TENGs based on skin sensors fabricated through a straightforward dip-coating method. The TENG utilizing the composite as both the electrode and the triboelectric layer exhibits exceptional energy harvesting capabilities.
CONCLUSIONS AND PERSPECTIVES
In considering the limitations of M-TENGs for practical use, such as their sensitivity to environmental conditions, limited mechanical durability over long-term use, and the challenge of achieving efficient energy conversion in low-frequency energy harvesting scenarios, it is clear that while these devices present a revolutionary approach to energy harvesting and self-powered systems, challenges such as environmental stability, integration complexity, and scalability must be rigorously addressed. The future vision of enhancing M-TENGs for broader energy collection, increased device sensitivity, and comfortability in wearable applications necessitates a holistic approach to overcoming these barriers.
M-TENG for energy collection
Increase in output power
To further enhance the output power of M-TENGs, an in-depth exploration of nanoscale engineering could be pivotal. For instance, developing nanostructured MXene surfaces through techniques such as etching or templating can significantly amplify surface roughness, thereby increasing the effective contact area and enhancing the triboelectric effect. Additionally, integrating MXene with other nanomaterials possessing high dielectric constants might provide a synergistic effect, boosting electron transfer efficiency and overall power output. Layered structures, where MXene sheets are interspersed with other high-performance materials, could create multiple triboelectric interfaces, multiplying the energy harvesting capability. Furthermore, exploring the potential of MXene heterostructures, where MXene is combined with other 2D materials such as graphene or TMDs, might open new avenues for higher electrical outputs. These modifications would not only increase the output power but could also contribute to tuning TENGs for specific frequency ranges of mechanical energy, making them more versatile in capturing energy from various ambient sources.
Increase in stability
Enhancing the stability of M-TENGs involves several innovative approaches. One strategy could be developing hybrid composites where MXene is combined with materials known for their environmental resistance, such as graphene or certain ceramics. These composites would not only protect MXene from degradation but also maintain its triboelectric properties over time. Advanced encapsulation techniques using materials such as polymers or glass could shield MXene layers from environmental factors such as moisture and oxygen, which are known to cause oxidation and degradation. Implementing self-healing materials into the MXene matrix is another forward-thinking method. These materials could autonomously repair microcracks or wear, thus preserving the structural integrity and functionality of TENGs. Additionally, optimizing the mechanical properties of MXene, such as flexibility and tensile strength, through material engineering could make TENGs more resilient to mechanical stress, ensuring long-term stability even under frequent or intense usage. Such advancements in stability are crucial for the practical application of TENGs in real-world environments where they are exposed to various physical and environmental stressors.
Expand energy collection channels
Expanding the energy collection channels of M-TENGs involves diversifying the types of mechanical energy these devices can capture and convert. The future could see M-TENGs tailored to harness energy from a wider range of sources, including environmental vibrations, acoustic waves, and even thermal motions. For instance, developing MXene composites with piezoelectric or pyroelectric materials could enable TENGs to capture energy from temperature fluctuations or heat sources. Additionally, designing TENGs that can efficiently convert low-frequency vibrations, such as those found in infrastructure or vehicles, into electrical energy could open up new avenues for energy harvesting in industrial settings. Another promising direction is integrating M-TENGs with fluid dynamics, enabling them to capture energy from water flow, raindrops, or wind movements. This approach could be particularly beneficial in remote or oceanic environments where wind and water are abundant. Innovations in design, such as flexible or foldable structures, could make TENGs more adaptable to different environmental conditions and surfaces, thereby broadening their applicability. Furthermore, hybrid energy systems that combine TENGs with other renewable energy technologies such as solar or wind power could create more comprehensive and efficient energy harvesting networks, capable of operating under various environmental conditions.
M-TENG's application
Increase in device sensitivity
Increasing the sensitivity of M-TENG devices, especially in applications such as sensors and wearable technology, will likely focus on material engineering and device architecture. By manipulating the nanostructure of MXene, such as creating thinner layers or specific patterns at the nanoscale, the surface properties can be finely tuned for enhanced responsiveness to mechanical stimuli. Incorporating MXene into composites with other sensitive materials such as conductive polymers or piezoelectric nanomaterials could produce devices that respond to even slight mechanical pressures, vibrations, or movements. This could be particularly impactful in wearable technology, where M-TENGs could monitor subtle physiological signals such as pulse, breathing, or muscle movements. Enhancing sensitivity also involves optimizing the interface between MXene layers and other components of the device to ensure efficient signal transduction. Advances in microfabrication techniques could create devices with intricate structures and high surface area-to-volume ratios, further improving their sensitivity. In the realm of sensor technology, this heightened sensitivity could lead to the development of highly responsive environmental monitors, capable of detecting minute changes in physical parameters, including pressure, humidity, or temperature. Such developments would greatly expand the potential uses of M-TENGs in various fields, including health monitoring, environmental sensing, and intelligent systems.
Increase in device comfortability
Enhancing the comfortability of M-TENG devices, particularly in wearable applications, will be a key development area. This can be achieved by integrating soft, flexible materials that conform to the body contours. Future advancements may include the development of ultra-thin, breathable MXene composites that maintain their triboelectric properties while offering a soft, fabric-like feel. This would involve exploring biocompatible materials that are gentle on the skin and avoid irritation, making them suitable for continuous wear. Additionally, the design of these devices could be optimized to reduce bulkiness and improve flexibility, allowing them to seamlessly integrate into everyday clothing or wearable accessories. Another aspect could be developing stretchable electronic circuits and connectors that maintain functionality even when stretched, twisted, or bent, which is essential for wearables that need to accommodate diverse body movements. Research into self-cooling materials or structures that dissipate heat could also contribute to increased comfort, especially for devices worn close to the skin for extended periods.
Expand application channels
Expanding the application channels of M-TENGs involves exploring new sectors and use cases where their unique properties can be beneficial. Future developments could see these devices being incorporated into smart city infrastructure, such as embedding them in roads or buildings to harness energy from pedestrian traffic or environmental vibrations. In healthcare, potential for M-TENGs in self-powered medical devices or implants exists, where they could power sensors that monitor health metrics or even deliver drug doses. Another promising area is in the field of robotics and artificial intelligence, where TENGs could provide a sustainable power source for sensors and small robotic components. Additionally, the automotive industry could benefit from integrating TENGs into vehicles for energy harvesting from vibrations and movements, contributing to the energy efficiency of electric vehicles. There is also potential for application in environmental monitoring, where M-TENGs could be used in remote or inaccessible locations to power sensors that track ecological changes or pollution levels. These expansions would not only highlight the versatility of MXene materials in various technological applications but also contribute to developing more sustainable and energy-efficient systems across diverse industries.
DECLARATIONS
Authors’ contributions
Searched a large amount of literature, wrote the manuscript, and obtained copyright licenses for all cited images in the manuscript: Zhao Z
Provide the research direction and funding support: Wang N, Cao X
Availability of data and materials
Not applicable.
Financial support and sponsorship
This work was funded by the National Key R&D Program of China. (2023YFF0612804), the National Natural Science Foundation of China (NSFC No. 51873020), and the University Basic Scientific Research Business Fee (No. FRF-MP-20-38).
Conflicts of interest
All authors declared that there are no conflicts of interest.
Ethical approval and consent to participate
Not applicable.
Consent for publication
Not applicable.
Copyright
© The Author(s) 2024.
REFERENCES
1. Zhou C, Zhao X, Xiong Y, et al. A review of etching methods of MXene and applications of MXene conductive hydrogels. Eur Polym J 2022;167:111063.
2. Lamiel C, Hussain I, Warner JH, Zhang K. Beyond Ti-based MXenes: a review of emerging non-Ti based metal-MXene structure, properties, and applications. Mater Today 2023;63:313-38.
3. Tawalbeh M, Mohammed S, Al-Othman A, Yusuf M, Mofijur M, Kamyab H. MXenes and MXene-based materials for removal of pharmaceutical compounds from wastewater: critical review. Environ Res 2023;228:115919.
4. Chang L, Xiao X. The review of MXenes for osmotic energy harvesting: synthesis and properties. Diam Relat Mater 2023;136:109971.
5. Guo Q, Guo J, Chen H, et al. Multi-functional graphene/leather for versatile wearable electronics. J Mater Chem A 2023;11:11773-85.
6. Zhou J, Chen H, Zhou P, et al. Ti3C2Tx MXene nanosheet-functionalized leathers for versatile wearable electronics. ACS Appl Nano Mater 2023;6:18150-64.
7. Zhao W, Zhou H, Li W, Chen M, Zhou M, Zhao L. An environment-tolerant ion-conducting double-network composite hydrogel for high-performance flexible electronic devices. Nanomicro Lett 2024;16:99.
10. Shakya J, Kang MA, Li J, et al. 2D MXene electrochemical transistors. Nanoscale 2024;16:2883-93.
11. Natu V, Barsoum MW. MXene surface terminations: a perspective. J Phys Chem C 2023;127:20197-206.
12. Sharma S, Kumar A, Ebenso EE. MXenes and MXene-based nanomaterials for corrosion protection. Mater Lett 2023;335:133789.
13. Athavale S, Micci-Barreca S, Arole K, et al. Advances in the chemical stabilization of MXenes. Langmuir 2023;39:918-28.
14. Mi Y, Lu Y, Shi Y, et al. Biodegradable polymers in triboelectric nanogenerators. Polymers 2022;15:222.
15. Zhao Z, Lu Y, Mi Y, et al. Adaptive triboelectric nanogenerators for long-term self-treatment: a review. Biosensors 2022;12:1127.
16. Mao R, Zhang D, Wang Z, et al. Deep-learning-assisted low-cost flexible cotton yarn-based triboelectric nanogenerator for ultra-sensitive human-computer merging interfaces. Nano Energy 2023;111:108418.
17. Xu Z, Zhang D, Cai H, Yang Y, Zhang H, Du C. Performance enhancement of triboelectric nanogenerators using contact-separation mode in conjunction with the sliding mode and multifunctional application for motion monitoring. Nano Energy 2022;102:107719.
18. Lu Z, Jia C, Yang X, et al. A flexible TENG based on micro-structure film for speed skating techniques monitoring and biomechanical energy harvesting. Nanomaterials 2022;12:1576.
19. Lu Y, Li X, Ping J, He J, Wu J. A flexible, recyclable, and high-performance pullulan-based triboelectric nanogenerator (TENG). Adv Mater Technol 2020;5:1900905.
20. Lin YC, Rinawati M, Chang LY, et al. A non-invasive wearable sweat biosensor with a flexible N-GQDs/PANI nanocomposite layer for glucose monitoring. Sensor Actuat B Chem 2023;383:133617.
21. Chen J, Wang P, Li J, et al. Self-powered antifouling UVC pipeline sterilizer driven by the discharge stimuli based on the modified freestanding rotary triboelectric nanogenerator. Nano Energy 2022;95:106969.
22. He S, Gui Y, Wang Y, Yang J. A self-powered β-Ni(OH)2/MXene based ethanol sensor driven by an enhanced triboelectric nanogenerator based on β-Ni(OH)2@PVDF at room temperature. Nano Energy 2023;107:108132.
23. Chen B, Wang ZL. Toward a new era of sustainable energy: advanced triboelectric nanogenerator for harvesting high entropy energy. Small 2022;18:e2107034.
24. Zhao Z, Lu Y, Mi Y, Meng J, Cao X, Wang N. Structural flexibility in triboelectric nanogenerators: a review on the adaptive design for self-powered systems. Micromachines 2022;13:1586.
25. Lu Y, Wu T, Ma Z, et al. Integration of flexible supercapacitors with triboelectric nanogenerators: a review. Batteries 2023;9:281.
26. Zhao Z, Zhu Q, Lu Y, Mi Y, Cao X, Wang N. Chemical sensor based on piezoelectric/triboelectric nanogenerators: a review of the modular design strategy. Chemosensors 2023;11:304.
27. Lu Y, Mi Y, Wu T, Cao X, Wang N. From triboelectric nanogenerator to polymer-based biosensor: a review. Biosensors 2022;12:323.
28. Zhao Z, Lu Y, Mi Y, et al. Modular design in triboelectric sensors: a review on the clinical applications for real-time diagnosis. Sensors 2023;23:4194.
29. Seidi F, Arabi Shamsabadi A, Dadashi Firouzjaei M, et al. MXenes antibacterial properties and applications: a review and perspective. Small 2023;19:e2206716.
30. Li K, Li J, Zhu Q, Xu B. Three-dimensional MXenes for supercapacitors: a review. Small Methods 2022;6:e2101537.
31. Wang Y, Cao X, Wang N. Recent progress in piezoelectric-triboelectric effects coupled nanogenerators. Nanomaterials 2023;13:385.
32. Wang X, Qin Q, Lu Y, et al. Smart triboelectric nanogenerators based on stimulus-response materials: from intelligent applications to self-powered systems. Nanomaterials 2023;13:1316.
36. Amara U, Hussain I, Ahmad M, Mahmood K, Zhang K. 2D MXene-based biosensing: a review. Small 2023;19:e2205249.
37. Yin Z, He R, Xue H, et al. A bimetallic-activated MnO2 self-assembly electrode with a dual heterojunction structure for high-performance rechargeable zinc-air batteries. Energy Mater 2022;2:200021.
38. Liu D, Shen J, Jian Z, Cai X. Advanced 3D-structured electrode for potassium metal anodes. Energy Mater 2023;3:300028.
39. Arjun AM, Shinde M, Slaughter G. Application of MXene in the electrochemical detection of neurotransmitters: a review. IEEE Sensors J 2023;23:16456-66.
40. Liang J, Zhou D, Liu D, Song H. Applications of MXenes in photoelectrochemistry: a review. Mater Res Bull 2024;169:112536.
41. Jung S, Zafar U, Achary LSK, Koo CM. Ligand chemistry for surface functionalization in MXenes: a review. EcoMat 2023;5:e12395.
42. Gopalram K, Kapoor A, Kumar PS, Sunil A, Rangasamy G. MXenes and MXene-based materials for removal and detection of water contaminants: a review. Ind Eng Chem Res 2023;62:6559-83.
43. Noor U, Mughal MF, Ahmed T, et al. Synthesis and applications of MXene-based composites: a review. Nanotechnology 2023;34:262001.
44. Chen Y, Sun H, Guo J, et al. Research on carbon-based and metal-based negative electrode materials via DFT calculation for high potassium storage performance: a review. Energy Mater 2023;3:300044.
45. Shahzad U, Marwani HM, Saeed M, Asiri AM, Rahman MM. Two-dimensional MXenes as emerging materials: a comprehensive review. ChemistrySelect 2023;8:e202300737.
46. Chu YZ, Hoover M, Ward P, Lau KC. First-principles study of MXene properties with varying hydrofluoric acid concentration. iScience 2024;27:108784.
47. Yang W, Wang X, Chen P, Hu Y, Li L, Sun Z. On the controlled adhesive contact and electrical performance of vertical contact-separation mode triboelectric nanogenerators with micro-grooved surfaces. Nano Energy 2021;85:106037.
48. He Y, Tian J, Peng W, Huang D, Li F, He Y. On the contact electrification mechanism in semiconductor-semiconductor case by vertical contact-separation triboelectric nanogenerator. Nanotechnology 2023;34:295401.
49. Ji S, Fu T, Hu Y. Effect of surface texture on the output performance of lateral sliding-mode triboelectric nanogenerator. J Phys Conf Ser 2020;1549:042095.
50. Saeed A, Liu Y, Shah MZ, Wang L, Wang Q. Sliding mode lateral guidance and control of finless airship. J Aerosp Eng 2022;35:04021131.
51. Padhan A, Hajra S, Sahu M, Nayak S, Joon Kim H, Alagarsamy P. Single-electrode mode TENG using ferromagnetic NiO-Ti based nanocomposite for effective energy harvesting. Mater Lett 2022;312:131644.
52. Mule AR, Dudem B, Patnam H, Graham SA, Yu JS. Wearable single-electrode-mode triboelectric nanogenerator via conductive polymer-coated textiles for self-power electronics. ACS Sustain Chem Eng 2019;7:16450-8.
53. Seo J, Hajra S, Sahu M, Kim HJ. Effect of cilia microstructure and ion injection upon single-electrode triboelectric nanogenerator for effective energy harvesting. Mater Lett 2021;304:130674.
54. Zhang Z, Bai Y, Xu L, et al. Triboelectric nanogenerators with simultaneous outputs in both single-electrode mode and freestanding-triboelectric-layer mode. Nano Energy 2019;66:104169.
55. Paosangthong W, Wagih M, Torah R, Beeby S. Textile-based triboelectric nanogenerator with alternating positive and negative freestanding woven structure for harvesting sliding energy in all directions. Nano Energy 2022;92:106739.
57. Luo J, Wang ZL. Recent progress of triboelectric nanogenerators: from fundamental theory to practical applications. EcoMat 2020;2:e12059.
58. Wang ZL. Triboelectric nanogenerator (TENG) - sparking an energy and sensor revolution. Adv Energy Mater 2020;10:2000137.
59. Jiang C, Li X, Yao Y, et al. A multifunctional and highly flexible triboelectric nanogenerator based on MXene-enabled porous film integrated with laser-induced graphene electrode. Nano Energy 2019;66:104121.
60. Liu Y, Li E, Yan Y, et al. A one-structure-layer PDMS/Mxenes based stretchable triboelectric nanogenerator for simultaneously harvesting mechanical and light energy. Nano Energy 2021;86:106118.
61. Rahman MT, Rana SMS, Salauddin M, et al. Silicone-incorporated nanoporous cobalt oxide and MXene nanocomposite-coated stretchable fabric for wearable triboelectric nanogenerator and self-powered sensing applications. Nano Energy 2022;100:107454.
62. Kim KN, Kim SY, Choi SH, et al. All-printed wearable triboelectric nanogenerator with ultra-charged electron accumulation polymers based on MXene nanoflakes. Adv Elect Mater 2022;8:2200819.
63. Li W, Lu L, Yan F, Palasantzas G, Loos K, Pei Y. High-performance triboelectric nanogenerators based on TPU/mica nanofiber with enhanced tribo-positivity. Nano Energy 2023;114:108629.
64. Tan X, Wang S, You Z, Zheng J, Liu Y. High performance porous triboelectric nanogenerator based on silk Fibroin@MXene composite aerogel and PDMS sponge. ACS Mater Lett 2023;5:1929-37.
65. Rana SMS, Rahman MT, Salauddin M, et al. Electrospun PVDF-TrFE/MXene nanofiber mat-based triboelectric nanogenerator for smart home appliances. ACS Appl Mater Interfaces 2021;13:4955-67.
66. Fan J, Yuan M, Wang L, Xia Q, Zheng H, Zhou A. MXene supported by cotton fabric as electrode layer of triboelectric nanogenerators for flexible sensors. Nano Energy 2023;105:107973.
67. Zhang Z, Yan Q, Liu Z, et al. Flexible MXene composed triboelectric nanogenerator via facile vacuum-assistant filtration method for self-powered biomechanical sensing. Nano Energy 2021;88:106257.
68. Cao W, Ouyang H, Xin W, et al. A Stretchable highoutput triboelectric nanogenerator improved by MXene liquid electrode with high electronegativity. Adv Funct Mater 2020;30:2004181.
69. Cai Y, Wang G, Mei Y, et al. Self-healable, super-stretchable and shape-adaptive triboelectric nanogenerator based on double cross-linked PDMS for electronic skins. Nano Energy 2022;102:107683.
70. Wang Z, Yao S, Wang S, et al. Self-powered energy harvesting and implantable storage system based on hydrogel-enabled all-solid-state supercapacitor and triboelectric nanogenerator. Chem Eng J 2023;463:142427.
71. Luo X, Zhu L, Wang Y, Li J, Nie J, Wang ZL. A flexible multifunctional triboelectric nanogenerator based on MXene/PVA hydrogel. Adv Funct Mater 2021;31:2104928.
72. Liu J, Zhang L, Wang N, Li C. Highly stretchable and transparent triboelectric nanogenerator based on multilayer structured stable electrode for self-powered wearable sensor. Nano Energy 2020;78:105385.
73. Xing C, Tian Y, Yu Z, Li Z, Meng B, Peng Z. Cellulose nanofiber-reinforced MXene membranes as stable friction layers and effective electrodes for high-performance triboelectric nanogenerators. ACS Appl Mater Interfaces 2022;14:36741-52.
74. Cao VA, Kim M, Lee S, et al. Chemically modified MXene nanoflakes for enhancing the output performance of triboelectric nanogenerators. Nano Energy 2023;107:108128.
75. Anwer S, Umair Khan M, Mohammad B, et al. Engineering of electrodes with 2D Ti3C2Tx-MXene sheets and chloride salt for robust and flexible high electrical power triboelectric nanogenerator. Chem Eng J 2023;470:144281.
76. Yun J, Park J, Ryoo M, Kitchamsetti N, Goh TS, Kim D. Piezo-triboelectric hybridized nanogenerator embedding MXene based bifunctional conductive filler in polymer matrix for boosting electrical power. Nano Energy 2023;105:108018.
77. Jiang C, Wu C, Li X, et al. All-electrospun flexible triboelectric nanogenerator based on metallic MXene nanosheets. Nano Energy 2019;59:268-76.
78. Song Z, Li W, Kong H, et al. Enhanced energy harvesting performance of triboelectric nanogenerator via efficient dielectric modulation dominated by interfacial interaction. Nano Energy 2022;92:106759.
79. Shao Y, Jin Z, Li J, Meng Y, Huang X. Evaluation of the electrochemical and expansion performances of the Sn-Si/graphite composite electrode for the industrial use. Energy Mater 2022;2:200004.
80. Li C, Zhang X, Zhu Y, et al. Modulating the lithiophilicity at electrode/electrolyte interface for high-energy Li-metal batteries. Energy Mater 2022;1:100017.
81. Huang T, Long M, Xiao J, Liu H, Wang G. Recent research on emerging organic electrode materials for energy storage. Energy Mater 2022;1:100009.
82. Yang C, Gao N, Wang X, et al. Stable and efficient seawater splitting on a porous phosphate-intercalated NiFe
83. Huang J, Hao Y, Zhao M, Li W, Huang F, Wei Q. All-fiber-structured triboelectric nanogenerator via one-pot electrospinning for self-powered wearable sensors. ACS Appl Mater Interfaces 2021;13:24774-84.
84. Deka BK, Hazarika A, Kwak MJ, et al. Triboelectric nanogenerator-integrated structural supercapacitor with in situ MXene-dispersed N-doped Zn-Cu selenide nanostructured woven carbon fiber for energy harvesting and storage. Energy Stor Mater 2021;43:402-10.
85. Hasan MM, Sadeque MSB, Albasar I, et al. Scalable fabrication of MXene-PVDF nanocomposite triboelectric fibers via thermal drawing. Small 2023;19:e2206107.
86. Bhatta T, Maharjan P, Cho H, et al. High-performance triboelectric nanogenerator based on MXene functionalized polyvinylidene fluoride composite nanofibers. Nano Energy 2021;81:105670.
87. Wang D, Zhang D, Tang M, et al. Ethylene chlorotrifluoroethylene/hydrogel-based liquid-solid triboelectric nanogenerator driven self-powered MXene-based sensor system for marine environmental monitoring. Nano Energy 2022;100:107509.
88. Sun X, Dong L, Liu Y, et al. Biomimetic PVA-PVDF-based triboelectric nanogenerator with MXene doping for self-powered water sterilization. Mater Today Nano 2023;24:100410.
89. Wang M, Wang X, Nan Y, et al. Droplet energy harvesting system based on MXene/SiO2 modified triboelectric nanogenerators. Chem Eng J 2023;477:146832.
90. Du Y, Wang X, Dai X, Lu W, Tang Y, Kong J. Ultraflexible, highly efficient electromagnetic interference shielding, and self-healable triboelectric nanogenerator based on Ti3C2T MXene for self-powered wearable electronics. J Mater Sci Technol 2022;100:1-11.
91. Rana SMS, Rahman MT, Zahed MA, et al. Zirconium metal-organic framework and hybridized Co-NPC@MXene nanocomposite-coated fabric for stretchable, humidity-resistant triboelectric nanogenerators and self-powered tactile sensors. Nano Energy 2022;104:107931.
92. Li K, Zhang D, Zhang H, et al. Triboelectric nanogenerators based on super-stretchable conductive hydrogels with the assistance of deep-learning for handwriting recognition. ACS Appl Mater Interfaces 2023;15:32993-3002.
93. Zhang H, Zhang D, Wang Z, et al. Ultrastretchable, self-healing conductive hydrogel-based triboelectric nanogenerators for human-computer interaction. ACS Appl Mater Interfaces 2023;15:5128-38.
94. Hao Y, Zhang Y, Mensah A, Liao S, Lv P, Wei Q. Scalable, ultra-high stretchable and conductive fiber triboelectric nanogenerator for biomechanical sensing. Nano Energy 2023;109:108291.
95. Chen Y, Tong W, Wang X, Zhang P, Wang S, Zhang Y. MXene effectively enhances the electron-withdrawing (EW) ability and dielectric properties of PVDF-TrFE nanofibers for triboelectric nanogenerators. Colloids Surf Physicochem Eng Asp 2023;664:131172.
96. Wang D, Zhang D, Yang Y, Mi Q, Zhang J, Yu L. Multifunctional latex/polytetrafluoroethylene-based triboelectric nanogenerator for self-powered organ-like mxene/metal-organic framework-derived CuO nanohybrid ammonia sensor. ACS Nano 2021;15:2911-9.
97. Wang D, Zhang D, Chen X, Zhang H, Tang M, Wang J. Multifunctional respiration-driven triboelectric nanogenerator for self-powered detection of formaldehyde in exhaled gas and respiratory behavior. Nano Energy 2022;102:107711.
98. Wang D, Zhang D, Tang M, et al. Rotating triboelectric-electromagnetic nanogenerator driven by tires for self-powered MXene-based flexible wearable electronics. Chem Eng J 2022;446:136914.
99. He W, Li S, Bai P, et al. Multifunctional triboelectric nanogenerator based on flexible and self-healing sandwich structural film. Nano Energy 2022;96:107109.
100. Zhi C, Shi S, Meng S, et al. A biocompatible and antibacterial all-textile structured triboelectric nanogenerator for self-powered tactile sensing. Nano Energy 2023;115:108734.
101. Ghosh K, Iffelsberger C, Konečný M, Vyskočil J, Michalička J, Pumera M. Nanoarchitectonics of triboelectric nanogenerator for conversion of abundant mechanical energy to green hydrogen. Adv Energy Mater 2023;13:2203476.
102. Nan Y, Wang X, Xu H, et al. Synergistic effects of charge transport and trapping in tribomaterials for boosted triboelectric nanogenerators. Nano Energy 2023;110:108345.
Cite This Article
Export citation file: BibTeX | RIS
OAE Style
Zhao Z, Cao X, Wang N. Beyond energy harvesting: a review on the critical role of MXene in triboelectric nanogenerator. Energy Mater 2024;4:400035. http://dx.doi.org/10.20517/energymater.2023.121
AMA Style
Zhao Z, Cao X, Wang N. Beyond energy harvesting: a review on the critical role of MXene in triboelectric nanogenerator. Energy Materials. 2024; 4(3): 400035. http://dx.doi.org/10.20517/energymater.2023.121
Chicago/Turabian Style
Zhao, Zequan, Xia Cao, Ning Wang. 2024. "Beyond energy harvesting: a review on the critical role of MXene in triboelectric nanogenerator" Energy Materials. 4, no.3: 400035. http://dx.doi.org/10.20517/energymater.2023.121
ACS Style
Zhao, Z.; Cao X.; Wang N. Beyond energy harvesting: a review on the critical role of MXene in triboelectric nanogenerator. Energy Mater. 2024, 4, 400035. http://dx.doi.org/10.20517/energymater.2023.121
About This Article
Special Issue
Copyright
Data & Comments
Data
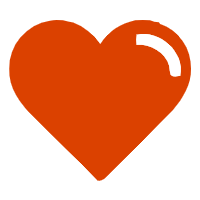

Comments
Comments must be written in English. Spam, offensive content, impersonation, and private information will not be permitted. If any comment is reported and identified as inappropriate content by OAE staff, the comment will be removed without notice. If you have any queries or need any help, please contact us at support@oaepublish.com.